A Comprehensive Guide to Editing The Electron Configuration In Ionic Bonding Section Review
Below you can get an idea about how to edit and complete a Electron Configuration In Ionic Bonding Section Review hasslefree. Get started now.
- Push the“Get Form” Button below . Here you would be brought into a dashboard allowing you to make edits on the document.
- Pick a tool you require from the toolbar that emerge in the dashboard.
- After editing, double check and press the button Download.
- Don't hesistate to contact us via [email protected] regarding any issue.
The Most Powerful Tool to Edit and Complete The Electron Configuration In Ionic Bonding Section Review
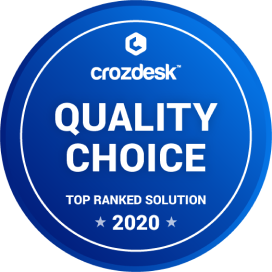
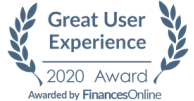
Complete Your Electron Configuration In Ionic Bonding Section Review At Once
Get FormA Simple Manual to Edit Electron Configuration In Ionic Bonding Section Review Online
Are you seeking to edit forms online? CocoDoc is ready to give a helping hand with its comprehensive PDF toolset. You can utilize it simply by opening any web brower. The whole process is easy and quick. Check below to find out
- go to the free PDF Editor Page of CocoDoc.
- Drag or drop a document you want to edit by clicking Choose File or simply dragging or dropping.
- Conduct the desired edits on your document with the toolbar on the top of the dashboard.
- Download the file once it is finalized .
Steps in Editing Electron Configuration In Ionic Bonding Section Review on Windows
It's to find a default application able to make edits to a PDF document. However, CocoDoc has come to your rescue. Examine the Manual below to form some basic understanding about ways to edit PDF on your Windows system.
- Begin by downloading CocoDoc application into your PC.
- Drag or drop your PDF in the dashboard and conduct edits on it with the toolbar listed above
- After double checking, download or save the document.
- There area also many other methods to edit PDF forms online, you can check this page
A Comprehensive Manual in Editing a Electron Configuration In Ionic Bonding Section Review on Mac
Thinking about how to edit PDF documents with your Mac? CocoDoc has come to your help.. It allows you to edit documents in multiple ways. Get started now
- Install CocoDoc onto your Mac device or go to the CocoDoc website with a Mac browser. Select PDF paper from your Mac device. You can do so by hitting the tab Choose File, or by dropping or dragging. Edit the PDF document in the new dashboard which provides a full set of PDF tools. Save the paper by downloading.
A Complete Instructions in Editing Electron Configuration In Ionic Bonding Section Review on G Suite
Intergating G Suite with PDF services is marvellous progess in technology, able to simplify your PDF editing process, making it quicker and more cost-effective. Make use of CocoDoc's G Suite integration now.
Editing PDF on G Suite is as easy as it can be
- Visit Google WorkPlace Marketplace and get CocoDoc
- set up the CocoDoc add-on into your Google account. Now you are more than ready to edit documents.
- Select a file desired by clicking the tab Choose File and start editing.
- After making all necessary edits, download it into your device.
PDF Editor FAQ
How does a multiple bond behave as a single bond?
Chemical bonding, any of the interactions that account for the association of atoms into molecules, ions, crystals, and other stable species that make up the familiar substances of the everyday world. When atoms approach one another, their nuclei and electrons interact and tend to distribute themselves in space in such a way that the total energy is lower than it would be in any alternative arrangement. If the total energy of a group of atoms is lower than the sum of the energies of the component atoms, they then bond together and the energy lowering is the bonding energy.crystal bondingDifferent types of bonding in crystals.Encyclopædia Britannica, Inc.The ideas that helped to establish the nature of chemical bonding came to fruition during the early 20th century, after the electron had been discovered and quantum mechanics had provided a language for the description of the behaviour of electrons in atoms. However, even though chemists need quantummechanics to attain a detailed quantitative understanding of bond formation, much of their pragmaticunderstanding of bonds is expressed in simple intuitive models. These models treat bonds as primarily of two kinds—namely, ionic and covalent. The type of bond that is most likely to occur between two atoms can be predicted on the basis of the location of the elements in the periodic table, and to some extent the properties of the substances so formed can be related to the type of bonding.A key concept in a discussion of chemical bonding is that of the molecule. Molecules are the smallest units of compounds that can exist. One feature of molecules that can be predicted with reasonable success is their shape. Molecular shapes are of considerable importance for understanding the reactions that compounds can undergo, and so the link between chemical bonding and chemical reactivity is discussed briefly in this article.Although simple models of bonding are useful as rules of thumb for rationalizing the existence of compounds and the physical and chemical properties and structures of molecules, they need to be justified by appealing to more-sophisticated descriptions of bonding. Moreover, there are some aspects of molecular structure that are beyond the scope of the simple theories. To achieve this insight, it is necessary to resort to a fully quantum mechanical description. In practice, these descriptions entail heavy reliance on computers. Such numerical approaches to the chemical bond provide important information about bonding.This article begins by describing the historical evolution of the current understanding of chemical bonding and then discusses how modern theories of the formation of chemical bonds have emerged and developed into a powerful description of the structure of matter. After the historical introduction, qualitative models of bonding are discussed, with particular attention given to the formation of ionic and covalent bonds and the correlation of the latter with molecular shapes. The more-sophisticated quantum mechanical approaches to bond formation are then described, followed by a survey of a number of special cases that raise interesting problems or lead to important insights.For a detailed discussion of the structure and properties of atoms, see atom. Chemical compounds are surveyed in the article chemical compound, and the elements are described in the article chemical element.Historical reviewAtomic structure and bondingBonds between atomsIt has been shown that, for reasons related to the energy requirements for electron removal or addition, only the electrons invalence shells play a significant role in the formation of bonds between atoms. Henceforth this article will concentrate on these electrons alone.Lewis introduced the conventions of representing valence electrons by dots arranged around the chemical symbol of the element, as in H· and Na·, and of discussing bond formation as the transfer of dots from one symbol to another. This seemingly simplistic device turns out to be very useful for establishing the characteristics of chemical bonds and will be examined in this section.The formation ofionic bondsLewis formulation of an ionic bondIn Lewis terms, the formation of an ionic bond stems from the transfer ofelectrons from one atom to another. When such a transfer occurs, all the valence electrons on the more electropositive element (from one of the first three groups on the left in the periodic table) are removed to expose the core of the atom. The electrons so released are accepted into the empty orbitals of the valence shell of the more electronegativeatom (typically from the groups immediately to the left of the noble gases); the valence shell is thereby filled. Thus, the formation of the ionic compoundsodium chloride can be represented by the following process:The formation of aluminum oxide (alumina) involves selecting enough aluminum and oxygen atoms to ensure that all the electrons released by the aluminum atoms (three from each one) are accommodated by oxygen atoms (each of which can accept two electrons):(The numbers of atoms required to balance the electrons donated and accepted is indicated by the chemical formula Al2O3for aluminum oxide.)That the transfer of electrons represented by these diagrams leads to a lowering of energy can be checked by assessing the energies associated with them. There is more to the process than a straightforward consideration of ionization energyand electron affinity. The ionization energy of sodium is larger than the electron affinity of chlorine, so energy is required to remove an electron from a sodium atom and attach it to a chlorine atom. That is, at first sight it appears that the total energy of a Na+ion and a Cl−ion is greater than that of a sodium atom and a chlorine atom. If that were the case, then it would be hard to understand how sodium chloride could be a stable species relative to a gas of sodium and chlorine atoms.There are in fact two errors in such a simple approach. First, the argument has ignored the favourable energy of interaction between the cation and the anion. The net energy of formation of a Na+ion and a Cl−ion is the sum of three terms. The first is the energy investment needed to ionize a sodium atom. The second is a somewhat smaller energy that is released when the electron from the sodium atom attaches to a chlorine atom. At this stage, the net energy change is positive, indicating a higher energy than for the two atoms. However, because there is an attraction between opposite charges, there is a further release of energy as a result of the interaction of the two ions. This additional favourable contribution to the energy varies with the separation of the ions and strengthens as the two ions approach one another. Thus, at large separations the neutral atoms have the lowest energy, but as the two atoms are brought together a point is reached at which the lowest total energy is obtained if an electron transfers from the sodium atom to the chlorine atom. At this distance, and at shorter distances, Na+Cl−is the lower-energy species.The second feature omitted from the argument is that anionic compounddoes not consist of an isolatedcationandanion. An ionic compound is typically a solid formed from an array of alternating cations and anions. The packing of ions together and their electrostatic interactions with one another account for the typical features of ionic compounds—namely, their brittleness and high melting points. Moreover, when studying the stability of such compounds, one should more appropriately consider the energy changes associated with their formation from the elements in their standard state (such as solid metallic sodium and gaseous chlorine molecules) than from a gas of atoms of the elements.The Born-Haber cycleThe analysis of the formation of an ionic compound from its elements is commonly discussed in terms of a Born-Haber cycle, which breaks the overall process into a series of steps of known energy. The Born-Haber cycle for the formation ofsodium chloride is shown in Figure 5. At the start of the cycle, the elements are considered to be in the form in which they exist at normal pressure and temperature. First, sodium metal is vaporized to a gas of sodium atoms. This step requires an input of energy known as the atomization energy of sodium metal. Next, the appropriate number of chlorine molecules (Cl2) are broken apart to provide a gas of chlorine atoms. This step also requires a considerable input of energy that is called the dissociation energy of chlorine. The origin of these two contributions to the energy can be clarified by considering metallic and covalent bonding in more detail (specifically, the lowering of energy that occurs when metallic or covalent bonds form); here they can be treated as empirical quantities. At this stage, an electron is removed from each sodium atom and attached to each chlorine atom. The ionization requires a considerable input of energy, and a fraction of that investment is recovered from the electron affinity of the chlorine atoms. Overall, however, there is a considerable increase in energy as compared to the two starting materials.Born-Haber cycleFigure 5: The Born-Haber cycle for the formation of solid sodium chloride from solid sodium and chlorine gas. The energies involved (strictly, the enthalpies) are expressed in kilojoules per mole.Encyclopædia Britannica, Inc.At this stage, the ions are allowed to come together to form acrystallinearray. This step releases a large quantity of energy called thelattice energy of the compound. Energy is released in the process of crystalformation because first a cation becomes surrounded by anions, then that cluster of anions becomes surrounded by cations, and so on. As a result of this packing, every cation has anions as neighbours, and every anion has cations around it, and there is a strong overall attractive interaction among the many ions of opposite charge in the crystal. For sodium chloride, the lattice energy is so great that more energy is released in this step than is required for all the preceding steps combined, and solid sodium chloride therefore has a lower energy than sodium metal and chlorine gas. It is for this reason that, when sodium reacts with chlorine, a large quantity of heat is released.Factors favouring ionic bondingA Born-Haber cycle gives an indication of the factors that favour ionic bonding. Overall, the lattice energy must be great enough to overcome the energy required for ion formation. It follows that only elements with reasonably low ionization energies can contribute, as cations, to ionic materials, for too large an ionization energy could not be recovered from the resulting lattice energy. In practice, this criterion means that onlymetallicelements are likely to form cations, and two elements are unlikely to form an ionic compound unless one of them is a metal. Moreover, the steep increase in ionization energy required to break into a closed shell precludes the loss of all but the valence electrons. Furthermore, no more than about three electrons per atom can be lost before the increase in ionization energy becomes prohibitive.It can also be seen from the Born-Haber cycle that elements will contribute anions to an ionic compound only if their electron affinity is positive or, at least, not too strongly negative. Elements with positive electron affinities are likely to form anions (as long as a metal is present). A negative electron affinity can be tolerated provided it is not too great and the additional energy investment can be recovered from a greater lattice energy. That is the reason why ionicoxides are so common: although energy is required to push the second electron on an oxygen atom to make an O2−ion, the resulting ion produces such a high lattice energy that the energy investment is overcome.Ionic bonding is likely to occur if the lattice energy of the compound is large, for a large lattice energy can compensate for some strongly demanding energy requirements, most notably for cation formation, earlier in the cycle. High lattice energies are achieved if the ions that form the lattice are small and highly charged, for small ions can pack together closely and interact strongly with one another. The O2−ion of oxides is small (oxygen lies well to the right in the periodic table) and is reasonably highly charged (it has two negative charges; three negative charges is about the limit for monatomic anions). As a result, ionic oxides are widely formed by metallic elements. Although it is conceivable that O3−ions could be formed if enough energy were provided to overcome the repulsion from the many electrons present in O2−, the necessary energy would not be recovered from the lattice energy, for O3−anions are so large that the lattice energy of any compound they would form would be small. Once again, the termination of electron gain at a noble gas configuration is not so much a sign of some magic stability of such a species but rather a consequence of the fact that after such a configuration has been attained there is insufficient opportunity for achieving a lower energy.The actual pattern in which cations and anions pack together is the one that results in the greatest lattice energy (that is, the greatest lowering of energy of the ions relative to the gas of ions). For further details on crystal arrangements, see crystal.Covalent bondsWhen none of the elements in a compound is a metal, no atoms in the compound have an ionization energy low enough for electron loss to be likely. In such a case, covalence prevails. As a general rule, covalent bonds are formed between elements lying toward the right in the periodic table (i.e., the nonmetals). Molecules of identical atoms, such as H2and buckminsterfullerene (C60), are also held together by covalent bonds.Lewis formulation of a covalent bondIn Lewis terms a covalent bond is a sharedelectron pair. The bond between a hydrogen atom and a chlorine atom inhydrogen chloride is formulated as follows:In a Lewis structure of acovalent compound, the shared electron pair between the hydrogen and chlorine ions is represented by a line. The electron pair is called abonding pair;the three other pairs of electrons on the chlorine atom are calledlone pairs and play no direct role in holding the two atoms together.Each atom in the hydrogen chloride molecule attains a closed-shell octet of electrons by sharing and hence achieves a maximum lowering of energy. In general, an incomplete shell means that some attracting power of a nucleus may be wasted, and adding electrons beyond a closed shell would entail the energetic disadvantage of beginning the next shell of the atom concerned. Lewis’soctet rule is again applicable and is seen to represent the extreme means of achieving lower energy rather than being a goal in itself.A covalent bond forms if the bonded atoms have a lower total energy than the widely separated atoms. The simplest interpretation of the decrease in energy that occurs when electrons are shared is that both electrons lie between two attracting centres (the nuclei of the two atoms linked by the bond) and hence lie lower in energy than when they experience the attraction of a single centre. This explanation, however, requires considerable modification to capture the full truth about bonding, and it will be discussed further below when bonding is considered in terms of quantum mechanics.Lewis structures of more complex molecules can be constructed quite simply by extending the process that has been described for hydrogen chloride. First, the valence electrons that are available for bonding are counted (2 × 1 + 6 = 8 inH2O, for example, and 4 + 4 × 7 = 32 incarbon tetrachloride, CCl4), and the chemical symbols for the elements are placed in the arrangement that reflects which are neighbours:Next, one bonding pair is added between each linked pair of atoms:The remaining electrons are then added to the atoms in such a way that each atom has a share in an octet of electrons (this is the octet-rule part of the procedure):Finally, each bonding pair is represented by a dash:(Note that Lewis structures do not necessarily show the actual shape of the molecule, only the topological pattern of their bonds.)In some older formulations of Lewis structures, a distinction was made between bonds formed by electrons that have been supplied by both atoms (as in H−Cl, where one shared electron can be regarded as supplied by the hydrogen atom and the other by the chlorine atom) and covalent bonds formed when both electrons can be regarded as supplied by one atom, as in the formation of OH−from O2−and H+. Such a bond was called acoordinate covalent bond or a dative bond and symbolized O → H−. However, the difficulties encountered in the attempt to keep track of the origin of bonding electrons and the suggestion that a coordinate covalent bond differs somehow from a covalent bond (it does not) have led to this usage falling into disfavour.Advanced aspects of Lewis structuresThe Lewis structures illustrated so far have been selected for their simplicity. A number of elaborations are given below.Multiple bondsFirst, an atom may complete its octet by sharing more than one pair of electrons with a bonded neighbour. Two shared pairs of electrons, represented by a double dash (=), form adouble bond. Double bonds are found in numerous compounds, includingcarbon dioxide:Three shared pairs of electrons are represented by a triple dash (≡) and form atriple bond. Triple bonds are found in, for example,carbon monoxide,nitrogen molecules, andacetylene, shown respectively as:A double bond is stronger than a single bond, and a triple bond is stronger than a double bond. However, a double bond is not necessarily twice as strong as a single bond, nor is a triple bond necessarily three times as strong. Quadruple bonds, which contain four shared pairs of electrons, are rare but have been identified in some compounds in which two metal atoms are bonded directly together.ResonanceThere is sometimes an ambiguity in the location of double bonds. This ambiguity is illustrated by the Lewis structure forozone (O3). The following are two possible structures:In such cases, the actual Lewis structure is regarded as a blend of these contributions and is written:The blending together of these structures is actually a quantummechanical phenomenon called resonance, which will be considered in more detail below. At this stage, resonance can be regarded as a blending process that spreads double-bond character evenly over the atoms that participate in it. In ozone, for instance, each oxygen-oxygen bond is rendered equivalent by resonance, and each one has a mixture of single-bond and double-bond character (as indicated by its length and strength).HypervalenceLewis structures and theoctet rulejointly offer a succinct indication of the type of bonding that occurs in molecules and show the pattern of single and multiple bonds between the atoms. There are many compounds, however, that do not conform to the octet rule. The most common exceptions to the octet rule are the so-called hypervalent compounds. These are species in which there are more atoms attached to acentral atom than can be accommodated by an octet of electrons. An example issulfur hexafluoride, SF6, for which writing a Lewis structure with six S−F bonds requires that at least 12 electrons be present around the sulfur atom:(Only the bonding electrons are shown here.) In Lewis terms, hypervalence requires theexpansion of the octet to 10, 12, and even in some cases 16 electrons. Hypervalent compounds are very common and in general are no less stable than compounds that conform to the octet rule.The existence of hypervalent compounds would appear to deal a severe blow to the validity of the octet rule and Lewis’s approach to covalent bonding if the expansion of the octet could not be rationalized or its occurrence predicted. Fortunately, it can be rationalized, and the occurrence of hypervalence can be anticipated. In simple terms, experience has shown that hypervalence is rare in periods 1 and 2 of the periodic table (through neon) but is common in and after period 3. Thus, the octet rule can be used with confidence for carbon, nitrogen, oxygen, and fluorine, but hypervalence must be anticipated thereafter. The conventional explanation of this distinction takes note of the fact that, in period-3 elements, the valence shell has n = 3, and this is the first shell in whichdorbitals are available. (As noted above, these orbitals are occupied after the 4s orbitals have been filled and account for the occurrence of the transition metals in period 4.) It is therefore argued that atoms of this and subsequent periods can utilize the empty d orbitals to accommodate electrons beyond an octet and hence permit the formation of hypervalent species.In chemistry, however, it is important not to allow mere correlations to masquerade as explanations. Although it is true that d orbitals are energetically accessible in elements that display hypervalence, it does not follow that they are responsible for it. Indeed, quantum mechanical theories of the chemical bond do not need to invoke d-orbital involvement. These theories suggest that hypervalence is probably no more than a consequence of the greater radii of the atoms of period-3 elements compared with those of period 2, with the result that a central atom can pack more atoms around itself. Thus, hypervalence is more a steric (geometric) problem than an outcome of d-orbital availability. How six atoms can be bonded to a central atom by fewer than six pairs of electrons is discussed below.Incomplete-octet compoundsLess common than hypervalent compounds, but by no means rare, are species in which an atom does not achieve an octet of electrons. Such compounds are called incomplete-octet compounds. An example is the compoundboron trifluoride, BF3, which is used as an industrial catalyst. The boron (B) atom supplies three valence electrons, and a representation of the compound’s structure is:The boron atom has a share in only six valence electrons. It is possible to write Lewis structures that do satisfy the octet rule.However, whereas in the incomplete octet structure the fluorine atoms have three lone pairs, in these resonance structures one fluorine atom has only two lone pairs, so it has partly surrendered an electron to the boron atom. This is energetically disadvantageous for such an electronegative element as fluorine (which is in fact the most electronegative element), and the three octet structures turn out to have a higher energy than the incomplete-octet structure. The latter is therefore a better representation of the actual structure of the molecule. Indeed, it is exactly because the BF3molecule has an incomplete-octet structure that it is so widely employed as a catalyst, for it can use the vacancies in the valence shell of the boron atom to form bonds to other atoms and thereby facilitate certain chemical reactions.Electron-deficient compoundsAnother type of exception to the Lewis approach to bonding is the existence of compounds that possess too few electrons for a Lewis structure to be written. Such compounds are called electron-deficient compounds. A prime example of an electron-deficient compound isdiborane, B2H6. This compound requires at least seven bonds to link its eight atoms together, but it has only 2 × 3 + 6 × 1 = 12 valence electrons, which is enough to form only six covalent bonds. Once again, it appears that, as in hypervalent compounds, the existence of electron-deficient compounds signifies that a pair of electrons can bond together more than two atoms. The discussion of the quantum mechanical theory of bonding below shows that this is indeed the case.A number of exceptions to Lewis’s theory of bonding have been catalogued here. It has further deficiencies. For example, the theory is not quantitative and gives no clue to how the strengths of bonds or their lengths can be assessed. In the form in which it has been presented, it also fails to suggest the shapes of molecules. Furthermore, the theory offers no justification for regarding anelectron pair as the central feature of a covalent bond. Indeed, there are species that possess bonds that rely on the presence of a single electron. (The one-electron transientspecies H2+is an example.) Nevertheless, in spite of these difficulties, Lewis’s approach to bonding has proved exceptionally useful. It predicts when the octet rule is likely to be valid and when hypervalence can be anticipated, and the occurrence of multiple bonds and the presence of lone pairs of electrons correlate with the chemical properties of a wide variety of species. Lewis’s approach is still widely used as a rule of thumb for assessing the structures and properties of covalent species, and modern quantum mechanical theories echo its general content.The following sections discuss how the limitations of Lewis’s approach can be overcome, first by extending the theory to account for molecular shapes and then by developing more thorough quantum mechanical theories of the chemical bond.Molecular shapes andVSEPR theoryThere is a sharp distinction betweenionic andcovalent bonds when the geometric arrangements of atoms in compounds are considered. In essence, ionic bonding is nondirectional, whereas covalent bonding is directional. That is, in ionic compounds there is no intrinsically preferreddirection in which a neighbour should lie for the strength of bonding to be maximized. In contrast, in a covalently bonded compound, the atoms adopt specific locations relative to one another, as in thetetrahedral arrangement of hydrogen atoms around the central carbon atom in methane, CH4, or theangular arrangement of atoms in H2O.The lack of directionality of ionic bonds stems from the isotropy (spherical symmetry) of the electrostatic forces between ions. As has already been pointed out, the result of this isotropy is that ionsstack together in the locations necessary to achieve the lowest energy and in this way give rise to the common packing patterns characteristic of many ionic solids. When deviations from stacking schemes are observed that seem to indicate that the ions are being held in certain orientations relative to their neighbours, it is a sign that covalent bonding is beginning to influence the structure of the solid and that the bonding is not purely ionic. This is the case, for example, in the compoundnickel arsenide (NiAs), which has a structure that suggests that a degree of covalent bonding is present (Figure 6). It is fully apparent in the structure of diamond (Figure 7), in which each carbon atom is in a tetrahedral position relative to its neighbour and in which the bonding is essentially purely covalent.nickel arsenideFigure 6: The crystal structure of nickel arsenide. This type of structure departs strongly from that expected for ionic bonding and shows the importance of covalence. There is also some direct nickel-nickel bonding that tends to draw the nickel atoms together.Encyclopædia Britannica, Inc.diamondFigure 7: The crystal structure of diamond. Each carbon atom is bonded covalently to four neighbours arranged tetrahedrally around the central atom. The structure is highly rigid.Encyclopædia Britannica, Inc.The rationalization of the structures adopted by purely ionic solids is essentially a straightforward exercise in the analysis of electrostatic interactions between ions. The problem of the structures of covalent compounds, both individual molecules, such as methane, and covalently bonded solids, such as diamond, is much more subtle, for it involves delving into the characteristics of the electron arrangements in individual atoms. Thus, if the formation of a covalent bond is regarded as corresponding to the accumulation of electrons in a particular region of an atom, then, to form a second bond, electrons can be accumulated into only certain parts of the atom relative to that first region of enhanced electron density. As a result, the bonds will lie in a geometric array that is characteristic of the atom. The remainder of this section focuses on this problem, but a detailed quantum mechanical analysis is required for a full understanding of the matter.The theory of molecular shape known as valence-shell electron-pair repulsion (VSEPR) theory grew out of Lewis’s theory, and, like that approach to bonding, VSEPR focuses on the role of electron pairs. It stems from the work of the British chemists H.M. Powell and Nevil V. Sidgwick in the 1940s and was extensively developed by R.J. Gillespie in Canada and Ronald S. Nyholm in London during the 1960s. As such, it postdates quantum mechanical theories of bonding and shape but should be seen (as is so common a motivation in chemistry) as an attempt to identify the essential features of a problem and to formulate them into a simple qualitative procedure for rationalization and prediction.A Lewis structure, as shown above, is a topological portrayal of bonding in a molecule. It ascribes bonding influences toelectron pairs that lie between atoms and acknowledges the existence of lone pairs of electrons that do not participate directly in the bonding. The VSEPR theory supposes that all electron pairs, both bonding pairs and lone pairs, repel each other—particularly if they are close—and that the molecular shape is such as to minimize these repulsions. The approach is commonly applied to species in which there is an identifiable central atom (the oxygen atom in H2O, for instance), but it is straightforward to extend it to discussions of the local shape at any given atom in a polyatomic species.Applying VSEPR theory to simple moleculesThemethane molecule, CH4, can be used to illustrate the procedure for predicting molecular shape. The Lewis structure of this molecule ascribes four bonding electron pairs to the carbon atom (Figure 8). These pairs repel one another, and their separation is maximized if they adopt a tetrahedral dispositionaround the central carbon atom. A hydrogen atom is attached by each bonding pair, so it can be predicted that CH4is likely to be a tetrahedral species, which is in fact the case.methaneFigure 8: The structure of methane, CH4. This regular tetrahedral structure is explained in the VSEPR theory of molecular shape by supposing that the four pairs of bonding electrons (represented by the gray clouds) adopt positions that minimize their mutual repulsion.Encyclopædia Britannica, Inc.When applying VSEPR theory, attention is first focused on the electron pairs of thecentral atom, disregarding the distinction between bonding pairs and lone pairs. These pairs are then allowed to move around the central atom (at a constant distance) and to take up positions that maximize their mutual separations. As in the methane molecule, four pairs adopt a tetrahedral disposition. The arrangements adopted by two through six pairs are summarized in the table. At this stage, the atoms that are attached by the bonding pairs are introduced, and the shape of the molecule is reported on the basis of the arrangement of these atoms.Thewater molecule, H2O, provides a simple example. The oxygen atom has four electron pairs, so these pairs adopt a tetrahedral arrangement. Two of the pairs are bonding, and hydrogen atoms are attached to them. Hence, the molecule is angular. (Note that the shape of the molecule is determined by the disposition of the atoms, not the disposition of the electron pairs.) Theammonia molecule, NH3, has four electron pairs in a tetrahedral arrangement around the nitrogen atom; three of these pairs are used to bond hydrogen atoms, so the molecule is predicted to betrigonal pyramidal, with a lone pair in the apical position. Some of the names of the shapes of simple molecules are summarized in the table.electron pairs and molecular shapeVSEPR electron pair arrangements and associated molecular shapes.Encyclopædia Britannica, Inc.Theangle between electron pairs in a tetrahedral arrangement is 109.5°. However, although H2O is indeed angular and NH3is trigonal pyramidal, the angles between the bonds are 104° and 107°, respectively. In a sense, such close agreement is quite satisfactory for so simple an approach, but clearly there is more to explain. To account for variations in bond angle, it is supposed that electron pair repulsions are greatest between lone pairs, less between lone pairs and bonding pairs, and least between bonding pairs. The justification of this ordering has proved somewhat elusive; qualitatively it is presumed that lone pairs, being attached only to a single centre, spread over a greater volume than bonding pairs, which are pinned between two attracting centres. Whatever the reason may be, the order correlates quite well with observation. Thus, in H2O the two lone pairs move apart a little, and the two bonding pairs move away from them by closing the angle between one another. Likewise, in NH3the three bonding pairs move back from the single lone pair to minimize their interaction with it. As a result, the H−N−H bond angle decreases slightly. In each case, the predicted angle is less than the tetrahedral angle, as is observed experimentally.VSEPR theory is quite successful at predicting (or at least rationalizing) the overall shapes of molecules. Thus, the hypervalent species SF6(sulfur hexafluoride), with six bonding pairs, is predicted and found to be a regular octahedron, and PCl5(phosphoruspentachloride), with five bonding pairs, is predicted and found to be atrigonal bipyramid. The XeF4(xenon tetrafluoride) molecule is hypervalent with six electron pairs around the central xenon (Xe) atom. These pairs adopt anoctahedral arrangement. Four of the pairs are bonding pairs, and two are lone pairs. According to VSEPR theory, the repulsion between the lone pairs is minimized if they lie on opposite sides of the xenon atom, leaving the four equatorial pairs as bonding pairs.This analysis suggests that XeF4should be a planar species, which is found to be the case.Molecules withmultiple bondsThere are further rules in VSEPR theory that simplify the discussion of species with multiple bonds and of species in which resonance must be considered. An analysis of the shapes adopted by species with multiple bonds suggests that each multiple bond can be treated as a single “superpair” of electrons. This rule can be justified by considering the geometric shapes that stem from two atoms sharing two or more pairs of electrons (Figure 9). Thus, thesulfate ion, SO42−, for which a Lewis structure ischemical bondFigure 9: Double bonds. The geometric arrangement of atoms linked by two shared pairs of electrons in a double bond (top) can be simulated by treating the double bond as the result of the sharing of a single superpair of electrons (bottom).Encyclopædia Britannica, Inc.can be treated as having the equivalent of four pairs (two ordinary pairs and two superpairs) around the sulfur atom in a tetrahedral arrangement. All four pairs arebonding, so the ion is predicted to be a regular tetrahedron, which it indeed is. The same conclusion about the shape of the molecule would be drawn from another possible Lewis structure, in which each bond is single:The actual molecule is aresonance hybrid of these and related structures; but, as each one corresponds to the same geometry, no particular Lewis structure need be selected before one can make a prediction based on VSEPR theory. In other words,resonance does not affect the shapes of molecules.Molecules with nocentral atomExamples of the manner in which VSEPR theory is applied to species in which there is no central atom are provided byethane (C2H6),ethylene(C2H4), andacetylene (C2H2), the Lewis structures for which are, respectively, the following:In each case, consider the local environment of each carbon atom. In ethane there are four bonding pairs around each carbon atom, so every carbon atom is linked to its four neighbours (one carbon atom and three hydrogen atoms) by a tetrahedral array of bonds. The bond angles in ethane are indeed all close to 109°. In ethylene each carbon atom possesses two ordinary bonding pairs (linking it to hydrogen atoms) and one superpair (linking it to the other carbon atom). These three pairs, and the corresponding bonds, adopt aplanar triangular arrangement, and the H−C−H and H−C=C angles are predicted to be close to 120°, as is found experimentally. It is less apparent from this analysis, but understandable once it is realized that the superpair is actually two shared pairs (Figure 9), that the ethylene molecule is predicted to be planar. Each carbon atom in an acetylene molecule has one bonding pair (to hydrogen) and one superpair (to the other carbon atom). The molecule is therefore expected to belinear, as is found in practice. The linearity of the molecule can be appreciated by referring to Figure 9.Limitations of the VSEPR modelThe VSEPR theory is simple yet powerful. Nevertheless, like any simplified model, it has its limitations. First, although it predicts that the bond angle in H2O is less than the tetrahedral angle, it does not make any attempt to predict the magnitude of the decrease. Second, the theory makes no predictions about the lengths of the bonds, which is another aspect of the shape of a molecule. Third, it ascribes the entire criterion of shape to electrostatic repulsions between bonding pairs, when in fact there are numerous contributions to the total energy of a molecule, and electrostatic effects are not necessarily the dominant ones. Fourth, the theory relies on some vague concepts, such as the difference in repelling effects of lone pairs and bonding pairs. There also are some species for which VSEPR theory fails. Nevertheless, despite these limitations and uncertainties, VSEPR theory is a useful rule of thumb and can be used with reasonable confidence for numerous species.Thepolarity of moleculesThere are three main properties of chemical bonds that must be considered—namely, their strength, length, and polarity. The polarity of a bond is the distribution of electrical charge over the atoms joined by the bond. Specifically, it is found that, while bonds between identical atoms (as in H2) are electrically uniform in the sense that both hydrogen atoms are electrically neutral, bonds between atoms of different elements are electrically inequivalent. Inhydrogen chloride, for example, the hydrogen atom is slightly positively charged whereas the chlorine atom is slightly negatively charged. The slight electrical charges on dissimilar atoms are called partial charges, and the presence of partial charges signifies the occurrence of a polar bond.The polarity of a bond arises from the relative electronegativities of the elements.Electronegativity, it will be recalled, is the power of an atom of an element to attract electronstoward itself when it is part of a compound. Thus, although a bond in a compound may consist of a shared pair of electrons, the atom of the more electronegative element will draw the shared pair toward itself and thereby acquire a partial negative charge. The atom that has lost its equal share in the bonding electron pair acquires a partial positive charge because its nuclear charge is no longer fully canceled by its electrons.The existence of equal but opposite partial charges on the atoms at each end of a heteronuclear bond (i.e., a bond between atoms of different elements) gives rise to anelectric dipole. The magnitude of this dipole is expressed by the value of itsdipolemoment, μ, which is defined as the product of the magnitude of the partial charges times their separation (essentially, the length of the bond). The dipole moment of a heteronuclear bond can be estimated from the electronegativities of the atoms A and B, χAand χB, respectively, by using the simple relationwhere D denotes the unit debye, which is used for reporting molecular dipole moments (1 D = 3.34 × 10−30coulomb·metre). Moreover, the negative end of the dipole lies on the more electronegative atom. If the two bonded atoms are identical, it follows that the dipole moment is zero and the bond is nonpolar.As the difference in electronegativity between two covalently bonded atoms increases, the dipolar character of the bond increases as the partial charges increase. When the electronegativities of the atoms are very different, the attraction of the more electronegative atom for the shared electron pair is so great that it effectively exercises complete control over them. That is, it has gained possession of the pair, and the bond is best regarded asionic. Ionic andcovalent bonding therefore can be regarded as constituting a continuum rather than as alternatives. This continuum can be expressed in terms of resonance by regarding a bond between atoms A and B as a resonance between a purely covalent form, in which the electrons are shared equally, and a purely ionic form, in which the more electronegative atom (B) has total control over the electrons:As the electronegativity difference increases, the resonance lies increasingly in favour of the ionic contribution. When the electronegativity difference is very large, as between an electropositive atom like sodium and an electronegative atom like fluorine, the ionic structure dominates the resonance, and the bonding can be regarded as ionic. Thus, as the electronegativity difference of the two bonded elements increases, a nonpolar bond gives way to a polar bond, which in turn becomes an ionic bond. There are, in fact, no purely ionic bonds, just as there are no purely covalent bonds; bonding is a continuum of types.Even a homonuclear bond, which is a bond between atoms of the same element (as inCl2), is not purely covalent, because a more accurate description would be in terms ofionic-covalent resonance:That the species is nonpolar despite the occurrence of ionic contributions stems from the equal contributions of the ionic structures Cl−Cl+and Cl+Cl−and their canceling dipoles. That Cl2is commonly regarded as a covalently bonded species stems from the dominant contribution of the structure Cl−Cl to this resonance mixture. In contrast, the valence bond theory wave function (see below Valence bond theory) of hydrogen chloride would be expressed as the resonance hybridIn this case, the two ionic structures would contribute different amounts (because the elements have different electronegativities), and the larger contribution of H+Cl−is responsible for the presence of partial charges on the atoms and the polarity of the molecule.Apolyatomic molecule will have polar bonds if its atoms are not identical. However, whether or not the molecule as a whole is polar (i.e., has a nonzero electric dipole moment) depends on the shape of the molecule. For example, the carbon-oxygen bonds incarbon dioxide are both polar, with the partial positive charge on the carbon atom and the partial negative charge on the more electronegative oxygen atom. The molecule as a whole is nonpolar, however, because the dipole moment of one carbon-oxygen bond cancels the dipole moment of the other, for the two bond dipole moments point in opposite directions in this linear molecule. In contrast, thewatermolecule is polar. Each oxygen-hydrogen bond is polar, with the oxygen atom bearing the partial negative charge and the hydrogen atom the partial positive charge. Because the molecule is angular rather than linear, the bond dipole moments do not cancel, and the molecule has a nonzero dipole moment.The polarity of H2O is of profound importance for the properties of water. It is partly responsible for the existence of water as a liquid at room temperature and for the ability of water to act as a solvent for many ionic compounds. The latter ability stems from the fact that the partial negative charge on the oxygen atom can emulate the negative charge of anions that surround each cation in the solid and thus help minimize the energy difference when the crystaldissolves. The partial positive charge on the hydrogen atoms can likewise emulate that of the cations surrounding the anions in the solid.The quantum mechanics of bondingIntermolecular forcesVarieties of solidsAdvanced aspects of chemical bondingMore About This TopicView All Media (28)Additional ReadingExternal WebsitesArticle HistoryArticle ContributorsKeep ExploringRelated Britannica ArticlesChemical associationChemical compoundChemistryElectronegativityHydrogen bondingIrving LangmuirMoleculeLinus PaulingTheory of resonanceVan der Waals forces
What is the amount of pollution caused by carbon nanotubes in an aquatic environment?
carbon nanotubes help to Environments as its absorb the pollutant from environmentSubscriber access provided by UNIV MASSACHUSETTS AMHERST Environmental Science & Technology is published by the American Chemical Society. 1155 Sixteenth Street N.W., Washington, DC 20036 Critical Review Adsorption Mechanisms of Organic Chemicals on Carbon Nanotubes Bo Pan, and Baoshan Xing Environ. Sci. Technol., 2008, 42 (24), 9005-9013• DOI: 10.1021/es801777n • Publication Date (Web): 13 November 2008 Downloaded from ACS Publications Home Page on March 19, 2009 More About This Article Additional resources and features associated with this article are available within the HTML version: • Supporting Information • Access to high resolution figures • Links to articles and content related to this article • Copyright permission to reproduce figures and/or text from this article Critical Review Adsorption Mechanisms of Organic Chemicals on Carbon Nanotubes BO PAN AND BAOSHAN XING* Department of Plant, Soil and Insect Sciences, University of Massachusetts, Amherst, Massachusetts 01003 Received July 8, 2008. Revised manuscript received October 15, 2008. Accepted October 16, 2008. Carbon nanotubes (CNTs) have drawn special research attention because of their unique properties and potential applications. This review summarizes the research progress of organic chemical adsorption on CNTs, and will provide useful information for CNT application and risk assessment. Adsorption heterogeneity and hysteresis are two widely recognized features of organic chemical-CNT interactions. However, because differentmechanismsmay act simultaneously, mainly hydrophobic interactions, π-π bonds, electrostatic interactions, and hydrogen bonds, the prediction of organic chemical adsorption on CNTs is not straightforward. The dominant adsorption mechanism is different for different types of organic chemicals (such as polar and nonpolar), thus different models may be needed to predict organic chemical-CNT interaction. Adsorption mechanisms will be better understood by investigating the effects of properties of both CNTs and organic chemicals along with environmental conditions. Another majorfactor affecting adsorption by CNTs istheir suspendability, which also strongly affects their mobility, exposure, and risk in the environment. Therefore, organic chemical-CNT interactions as affected by CNT dispersion and suspending merit further experimental research. In addition, CNTs have potential applications in water treatment due to their adsorption characteristics. Thus column and pilot studies are needed to evaluate their performance and operational cost. Introduction Carbon nanotubes (CNTs) have attracted special attention because of their unique properties, such as electrical conductivity, optical activity, and mechanical strength. This fascinating new class of materials has shown promising application inmany areas since its discovery. However, CNTs are being spread quickly in the environment because of their growing use (1, 2). Several studies indicate that they are toxic to organisms and human beings (3), and their presence in the environment affects the behavior of pollutants, such as heavy metals as reviewed by Rao et al. (4). Because of their hydrophobic surfaces, strong interactions between CNTs and organic chemicals are expected. Numerous studies suggest CNTs as effective adsorbents for organic chemicals in solidphase extraction and water treatment after compared with C18 (5, 6) and activated carbon (AC) (7, 8). This strong interaction also greatly alters the mobility, bioavailability, and environmental risk of organic chemicals (9, 10). In addition, because the structures of CNTs are well defined and their surfaces are relatively uniform in contrast to AC, CNTs are considered to be a good choice to study adsorption mechanisms. Therefore, the understanding of organic chemical-CNT interactions will provide important information on assessing CNT environmental risks and in exploring their applications. Nevertheless, research in this area is still fragmentary and not complete enough for making clear conclusions. An overview of research progress on the interactions between organic chemicals and CNTs is urgently needed. Thus, in this review, we first summarize general features of organic chemical adsorption on CNTs, and then discuss adsorption mechanisms in detail by probing into how the properties of CNTs and organic molecules, coupled with environmental conditions, affect adsorption of organic chemicals by CNTs. General Features of Organic Chemical Adsorption on CNTs Heterogeneous Adsorption. Most directly, heterogeneous adsorption indicates that organic chemical adsorption on CNTs could not be described using a single adsorption coefficient. If a single coefficient were used, significant error would occur when predicting the organic chemical-CNT interaction, and would consequently lead to a wrong conclusion regarding the environmental risk of both organic chemicals and CNTs. To date, various models have been applied to describe the adsorption of organic molecules on CNTs in aqueous phases, such as Freundlich (5, 6, 11), Langmuir (7, 8, 12, 13), BET (14), and Polanyi-Manes models (15, 16). Two reasons have been provided to explain the heterogeneous adsorption. The first is the presence of highenergy adsorption sites, such as CNT defects (17), functional groups (18), and interstitial and groove regions between CNT bundles (Figure S1 in the Supporting Information) (19). These adsorption sites commonly exist on as-grown CNTs (20), thus heterogeneous adsorption is a general feature. The second reason is condensation, such as surface and capillary condensation of gas or liquid adsorbates. Multilayer adsorption could occur when organic chemicals were adsorbed on CNT surfaces (21, 22). In this process, the first couple of layers interact with the surface, while molecules beyond the first two layers interact with each other. This process is called surface condensation. The energy of this process varies depending on the distance between the adsorbed molecules and the CNT surface, thus causing a distribution of adsorption energy. The inner pore of an open-end CNT or interstitial area in a CNT bundle forms a hollow column with both ends open. If the pore size follows the Kelvin-Laplace equation, * Corresponding author phone: 413-545-5212; fax: 413-545-3958; e-mail: [email protected]. 10.1021/es801777n CCC: $40.75 2008 American Chemical Society VOL. 42, NO. 24, 2008 / ENVIRONMENTAL SCIENCE & TECHNOLOGY 9 9005 Published on Web 11/13/2008 capillary condensation takes place andis responsible for loaddependent adsorption energy and heterogeneous adsorption (23, 24). Both the aforementioned reasons indicate distributed adsorption energy of adsorption sites. Organic chemicalsmay occupy high-energy adsorption sites first, and then spread to sites with lower energy (25). This hypothesis indicates concentration-dependent thermodynamics and kinetics. Concentration-dependent thermodynamics has been observed in laboratory studies for organic vapors (11, 23), but has not been studied for the aqueous phase. Researchers also tried to explain prolonged adsorption/desorption kinetics by heterogeneous adsorption sites (26, 27). However,more direct evidence would be concentration-dependent kinetics, which has not been reported. Hysteresis. Adsorption/desorption hysteresis was observed for small molecules (such as organic vapors of methane, ethylene, and benzene) as well as polymers (such as poly(aryleneethynylene)s) on CNTs (28). Hysteresis was presented as deviation of desorption curves from adsorption ones (29) and the absence of adsorbate in the supernatant when CNTs were repeatedly washed using organic solvents (30), buffers (31), or water (32). Real hysteresis is emphasized by conducting recovery tests, verification of equilibrium (29), or direct observation using transmission electronmicroscopy (TEM) and atomic force microscopy (30, 32). However, a lack of hysteresis was reported for butane (14), PAHs (9), and atrazine (15). Different hysteresis phenomena result in different opinions on CNT-related risk. For example, high adsorption capacity and reversible desorption of organic chemicals on CNTs imply the potential release of organic chemicals after intake by animals or human (9). In this case, CNTs act like pollutant collectors and thus pose high health risk. However, significant adsorption/desorption hysteresis can make CNTs pollutant sinks. This would result in decreased organic chemical mobility, bioavailability, and environmental risk (10). Therefore, proper understanding of hysteresis mechanisms is a key step toward assessing CNTled risk and application. Different mechanisms have been proposed to explain the hysteresis, e.g., the strong π-π coupling of benzene-ringcontaining chemicals with the CNT surface (30, 32) and capillary condensation (23). In addition, alteration of adsorbent structure or reorganization after adsorption has been widely accepted to explain desorption hysteresis for organic chemicals on soils/sediments (33). This explanation is applicable in organic chemical-CNT adsorption systems. For example, CNTs with adsorbed tetra-tert-butylphthalocyanine could be easily dispersed in CHCl3, while the pure CNTs could not (30). This result indicated that the π-π bonds between organic chemicals and CNTs disrupted Van der Waalsinteractions between CNTs andinhibited the formation of bundles. Therefore, the interference of CNT-CNT interactions by adsorption of organic chemicals results in different pathways between desorption and adsorption, which consequently induces hysteresis. The absence of adsorption/desorption hysteresis was previously observed, which is an exception to the aforementioned mechanisms. When CNTs had few particleparticle contacts (as shown by TEM images in ref 14), CNT bundles were hardly formed and no interstitial regions were available for butane adsorption. The pore condensation accounted for less than 1% of the uptake (14). Therefore, the lack of hysteresis was explained well by the morphology of CNTs during sorption process. In another system, the KHW (hexadecane-water distribution coefficient) normalized adsorption coefficients (K/KHW) of PAHs (9) were more than 1000 times lower than those of two estrogens containing phenolic groups (29). The adsorption of PAHs on CNTs may not be strong enough to disrupt or disassociate CNT bundles, and thus no hysteresis was observed (9). Multiple Mechanisms Acting Simultaneously. The outer surface of individual CNTs provides evenly distributed hydrophobic sites for organic chemicals. Hydrophobic interactions were emphasized in several studies that discussed protein (31), naphthalene (22), acidic herbicides (6), and streptavidin (34) adsorption on CNTs. If hydrophobic interactions are the only mechanism for the interactions between organic chemicals and CNTs, the adsorption can be predicted using the hydrophobic parameters of organic chemicals, such as KOW (octane-water distribution coef- ficient) or KHW. If this is the case, fate modeling on the environmental behavior of organic chemicals in the presence of CNTs would be straightforward. However, this is not true for most cases. For example, Chen et al. (35) reported poor correlations between the adsorption affinity and hydrophobicity of several aromatic derivatives. Furthermore, the KHW normalized adsorption coefficient varied more than 1000 times for several organic chemicals on CNTs (29). An analysis of literature data also failed to establish a general relationship between KOW and adsorption coefficients (Figure 1 and Figures S2, S3, and Table SI, Supporting Information). Thus, hydrophobic interactions cannot completely explain the interaction between organic chemicals and CNTs. Other mechanisms include π-π interactions (between bulk π systems on CNT surfaces and organic molecules with CdC double bonds or benzene rings), hydrogen bonds (because of the functional groups on CNT surfaces), and electrostatic interactions (because of the charged CNT surface) (36, 37). Different adsorption mechanisms respond to the change of environmental conditions differently, thus, the relative contribution of an individual mechanism to the overall adsorptionis ofmajorimportance to predict organic chemical adsorption on CNTs. However, present studies mostly emphasize the importance of individual mechanisms, but never propose a method to quantitatively determine the relative contribution of individualmechanisms. For example, the importance of π-π interactions was demonstrated by comparing the adsorption of several carefully selected organic chemicals on CNTs (38) or by comparing the adsorption FIGURE 1. Relationships between the adsorption coefficient (K) of organic chemicals on SWCNTs (a) or MWCNTs (b) and their KOW. K was calculated at the equilibrium concentration of 100 µg/L. The database for this analysis is provided in Table SI, Supporting Information. More analysis on detailed classification of CNTs is presented in Figures S1 and S2. No explicit relationship between K and KOW was observed indicating that hydrophobic interactions are not the only mechanism for the adsorption of organic chemicals on CNTs. 9006 9 ENVIRONMENTAL SCIENCE & TECHNOLOGY / VOL. 42, NO. 24, 2008 before and after a π-π system was interrupted (30). However, the authors did not further discuss the quantification of π-π bond contribution relative to the overall adsorption. The following method may be considered in order to reveal the relative contribution of a given mechanism: (1) Normalization of the sorption coefficient by KHW may screen out the hydrophobic effect, and thus investigators could focus on factors other than hydrophobicity (35). Although KOW has been widely used to describe hydrophobicity, it has been reported to be unsuitable to normalize adsorption coefficients because of the possible interaction between -OH on octanol with organic chemicals (29). Normalization of the adsorption coefficient by KHW is probably a better and more reliable method. (2) A comparison of the adsorption of various organic chemicals on a given type of CNTs will present important information about the contribution of different adsorption mechanisms. For example, sorption comparison of a series of nonpolar chemicals with the same KHW but different numbers of π electrons could reflect the contribution of π-π interactions. Adsorption of a given organic chemical on CNTs with different extents of oxidation or functionalization should be compared with great caution because oxidation or functionalization changes not only CNT functional groups, but also surface area, surfacecharge, andCNThydrophobicity. (3) More directly, sorption experiments could be conducted in organic solvents. A comparison of the adsorption of a given chemical on CNTs from organic solvents with different polarities could directly derive the relative contribution of hydrophobic effects or other mechanisms (Figure S4). Another benefit for studying adsorption in organic solvents is to ensure reliable detection by keeping the adsorbate concentration well above the detection limit due to high solubility in organic solvents. Adsorption Affected by CNT Properties Correlation of Adsorption with CNT Physical Properties. CNT surface areas are normally in the range of 290 ( 170 m2/g (mean ( standard error, Table SI) and are generally lower than that of ACs (39). But organic chemical adsorption on CNTs (especially on single-walled CNTs, SWCNTs) is comparable to or even higher than that on ACs (29). Thus, surface area may not be a direct parameter to predict organic chemical-CNT interactions. Su and Lu (7) attributed the higher adsorption of dissolved organic matter (DOM) on CNTs to larger average pore diameter and volume. However, in most studies, CNT porosity could not be applied to explain high adsorption. Decreased CNT diameter increases surface curvature, leading to increased number of multilayers (14), stronger adsorption (16, 40, 41), and increased separation of molecular species in a binary mixture (42). But for molecules with planar structures, adsorption increases with increased diameter because the flat surface results in better contact, such as tetracene (43) and benzene (44). The balance between these two opposite effects deserves further study. Therefore, it seems that neither CNT surface area and porosity, nor diameter alone, could be used to explain CNT adsorption characteristics completely. Adsorption that is affected by other CNT properties, such as morphology and functional groups, will be further addressed in the following sections. Morphology of CNTs. The CNT that contains one layer of a rolled graphite sheet is called SWCNT, whereas several SWCNTs with different diameters concentrically nested together is called multiwalled CNT (MWCNT). The distance between the layers of MWCNTs is too small for any organic molecule to fit into (45). CNTs tend to aggregate together as bundles because of Van der Waals interactions (19). Thus, for an ideal case, the available sorption sites of CNT bundles include the surface area, the interstitial and groove areas formed between the CNTs, and the inner pores of the tubes (Figure S1). External surface and groove areas are generally available for adsorption, but the interstitial and inner pores are not. For example, the external surface of SWCNT is the main area for naphthalene adsorption, but the inner pore sites are not due to the dimensional restrictions (22). On the other hand, molecules as big as enzymes were reported to enter the inner pores of CNTs with diameter 3-5 nm (46). The presence of amorphous carbon, functional groups, and metal catalysts could block the inner pores (16). The blocked inner pores can be opened up by acid treatment using HCl to eliminate metal catalysts located at the end of the CNTs (47), or using H2O2 (21), nitric acid (22), base (48), or heat treatment (563 K in ref 43) to remove the amorphous carbon. The reason for the unavailable interstitial sites is that no bundle was formed (14) or that the organic molecules are too large to fit into this area (45). Thus, the availability of sites for organic chemical adsorption on CNTs is highly dependent on CNT properties as well as their aggregation. Liu et al. (49) observed higher sorption of organic dyes on CNTs in water than in ethanol. However, they mostly discussed the different ionic states of the dyes in different solvents. As the authors presented in their TEM results, most of the dye-functionalized CNT showed debundled structure. Hence, the change of CNT aggregation could be another key factor for organic chemical adsorption. Another character of CNT morphology is related to the angle between the graphite plane and the tube axis, which determines the chirality of CNTs: zigzag, armchair, and chiral structures. The C-C bonds in graphite are all the same, but C-C bonds in CNTs are different by length and orientation to the tube axis (44). Thus both adsorption energy and the distance between organic chemicals and CNT surfaces could differ between zigzag and armchair tubes of a same curvature (diameter). However, no experiments have been conducted for organic chemical sorption on CNTs with different chiralities. Functional Groups of CNTs. CNTs possibly contain functional groups such as sOH, sCdO, and sCOOH depending on the synthetic procedure and purification process. Functional groups can also be intentionally added by oxidation (22) or removed by heat treatment (such as 2200 °C in ref 50). In a well controlled experiment, 3.3-14% surface oxides could be sequentially incorporated on CNTs using nitric acid (39). Ago et al. (51) quantified surface functional groups of acid-oxidized CNT using X-ray photoelectron spectroscopy, and presented that 5, 2, and 7% of the carbon atoms were sCsOs, sCdO, and sCOOs, respectively. The air-oxidized CNTs showed higher sCsOs (9%), but lower sCOOs (3%) content. Functionalization of CNTs is aimed for easy processing, but at the same time, their adsorption properties with organic chemicals can be altered greatly. Functional groups can change the wettability of CNT surfaces, and consequently make CNT more hydrophilic and suitable for the adsorption of relatively low molecular weight and polar compounds (13, 50, 52). On the other hand, functional groups may increase diffusional resistance (47) and reduce the accessibility and affinity of CNT surfaces for organic chemicals (39, 53). An overall view of the effect of CNT functional groups on organic molecule adsorption is summarized in Figure 2. In previous studies, hydrogen bonds (H-bond) have been discussed extensively to understand organic chemical sorption on ACs (54). However, several studies reported that increased oxygen-containing functional groups on ACs decreased the adsorption of chemicals which can form H-bonds (55). These opposite results call for further research on the H-bond mechanism. In addition, water molecules could also form H-bonds with functional groups on ACs, which will either compete with organic chemicals for adsorption sites (54) or form a three-dimensional cluster VOL. 42, NO. 24, 2008 / ENVIRONMENTAL SCIENCE & TECHNOLOGY 9 9007 and block the sorption sites nearby (56). Thus, the H-bond formation between water and AC functional groups could effectively decrease the sorption of organic chemicals. Therefore, the relative H-bond strength of water-ACs to organic chemicals-ACs determines the extent for adsorption of organic chemicals. Similar arguments apply for organic chemical sorption on CNTs. Although H-bonds are reported unlikely to be a primary interaction mechanism (36, 37), benzene ring on CNT surface may act as H-bond donor and form H-bonds with oxygen-containing functional groups on organic chemicals (57). In addition, H-bonds may play an important role for ionic chemical adsorption on oxidized CNTs. Clearly, further study is required to systematically evaluate H-bonds in organic chemical-CNT interactions. During CNT surface functionalization, purification, or exposure to oxidizing agents after release to the environment, CNTs will eventually be oxidized (39). Therefore, a better understanding of toxicology and adsorption properties of oxidized CNTs is important for CNT environmental risk assessment. Adsorption Mechanisms of Different types of Organic Chemicals on CNTs Molecular Morphology.Molecular size and shape determine the availability of different adsorption sites on CNTs. The discussion of the relative size between organic molecules and CNTs applies here, too. Specifically for organic chemicals, larger molecules have higher adsorption energies, and thus larger differences in molecular size result in better separation in a system with mixed chemicals (42). Linear hydrocarbons (42) and planar chemicals (49), especially linear planar chemicals (such as tetracene in ref 58), have a better contact with the CNT surface than other chemicals, and hence show stronger adsorption on CNTs. The bottleneck for the rate of organic chemical diffusion is also dependent on molecular size. An example is given by slow diffusion of water and ethanol as compared to that of n-hexane (26). Sorption of n-hexane on CNTs reached equilibrium in 20-30 min, while water and ethanol took more than 10 h. This phenomenonmay be partially explained by the higher hydrophobicity of hexane as the authors presented. However, their data could be reevaluated because the much higher sorption capacity (more than 10 times higher) for water and ethanol may indicate different availability of adsorption sites for chemicals with different sizes. For smaller molecules (water and ethanol), the diffusion into inner sites of CNTs can be the rate-limiting process, and could result in extremely low diffusivities. However, bigger molecules (hexane) have a much faster sorption rate because contribution of hexane adsorption in the inner pores is very low. Molecules, especially the larger ones, can twist themselves so that they match with the curvature surface, thus forming stable complexes with the CNTs (59-61). This molecular reorganization is possible because the adsorption energy can compensate the steric energy required for conformational changes of organic chemicals (29). Wang et al. (30) reported lower decomposition temperature for tetra-tert-butylphthalocyanines after adsorption on CNTs, which is a result of the increased internal energy. A similar result was also reported for L-phenylalanine sorption on CNTs (52), however, the authors attributed the reduced decomposition temperature to the catalysis of CNTs. The geometrical configurations of both chemicals and CNTs affect their interactions. It is reasonable to expect a significant effect of the geometrical configurations of both chemicals and CNTs on their interactions because the surface curvature of CNTs is comparable to the dimensions of organicmolecules. However, few studies have yet focused on conformational rearrangement of organic chemicals on CNTs. Functional Groups of Organic Chemicals. Each carbon atom in a CNT has a π electron orbit perpendicular to CNT surface (62). Therefore, organic molecules containing π electrons can form π-π bonds with CNTs, such as organic molecules with CdC double bonds or benzene rings, which has been confirmed by experimental data (36, 37, 63), molecular dynamic simulations (64-66), Raman band (58), Fourier transform infrared spectra (43), and nuclearmagnetic resonance spectra (28). Themost widely recognized influence of organic chemical functional groups on organic chemicalCNT interactions is on the electron-donor-acceptor (EDA) π-π interaction, i.e., the strength of π-π bond is greatly dependent on the functional groups attached to the benzenerings for organic chemicals (37). Because CNTs could be viewed as either electron-donors or acceptors, adsorption of either electron-acceptors (such as nitroaromatics in ref 35) or electron-donors (such as phenols in ref 53) on CNTs is expected to be stronger as compared to unsubstituted FIGURE 2. Adsorption properties as affected by CNT functional groups. This figure shows the general trend for the changes of CNT adsorption properties after different treatments. The surfaces of raw CNTs are hydrophobic as demonstrated by the strong preference for adsorption of hydrocarbons (such as hexane, benzene, and cyclohexane) over alcohols (such as ethanol, 2-propanol). Functionalization will lead to increased oxygen content, decreased surface area, and reduced adsorption of nonpolar hydrocarbons due to reduced hydrophobicity, and so do planar chemicals due to insufficient contact between CNT and the chemical. Graphitization will eliminate functional groups, and decrease the adsorption of polar chemicals, but will increase the adsorption of nonpolar and/or planar hydrocarbons. 9008 9 ENVIRONMENTAL SCIENCE & TECHNOLOGY / VOL. 42, NO. 24, 2008 aromatic hydrocarbons. In addition, the tendency of a molecule to accept or donate electrons also determines the strength of the π-π bond, as in the case with strong charge donors over weak charge donors (such as 2,3-dichloro-5,6- dicyano-1,4-benzoquinone over benzene in ref 65). The introduction of carboxylic groups to CNTs made CNTs electron acceptors. Thus increased adsorption of electron donors (such as phenanthrene in ref 43 and BTEX in ref 67), and decreased adsorption of electron acceptors (such as chlorophenol in ref 68) were observed. Therefore, quantification of EDA strength could provide a valuable parameter to predicting organic chemical-CNT interactions. Zhao et al. (65) used a charge transfer parameter, specifically, the total Mulliken charge number on several molecules, to quantitatively describe the ability of a certain molecule to donate or withdraw electrons. Sone et al. (69) proposed that aromatic compounds with a smaller gap between the highest occupied molecular orbital energy and the lowest unoccupied molecular orbital will have a higher affinity toward CNTs. Both methods seem to be promising to predict the strength of organic chemical-CNTinteractions. However, no information is available on how well these theories could explain experimental data. Additional laboratory experiments should be conducted. Functional groups also greatly determine organic chemical polarity. Because the predominant mechanisms are different for polar and nonpolar chemicals, predictions of their adsorption on CNTs require different models. For example, for polar organic chemicals, the adsorption tends to increase with increased CNT oxygen content because of the enhanced H-bond or EDAinteraction. However, for nonpolar chemicals, the adsorption may decrease with increased CNT oxygen content because of the depressed hydrophobic interaction (Figure S4). These opposite trends have not been examined extensively in literature, but could be very important for CNT applications. For example, if CNTs are to be used in water treatment, chemical-specific modification may be needed to improve treatment performance. For the same reason, chemical-specific models may also be needed to predict organic chemical-CNT interactions. The present data are too limited for further discussion on predictive models. Adsorption Affected by Environmental Conditions Environmental conditions have not been widely studied for their impact on organic chemical-CNT interactions. However, a limited number of studies in this area have demonstrated their importance. pH and Ionic Strength. For ionizable organic chemicals, the variation in pH can result in a change in chemical speciation, consequently altering their adsorption characteristics. Increased pH generally leads to increased ionization, solubility, and hydrophilicity, and thus decreased adsorption of natural organic matter (8, 70), resorcinol (53), and herbicides (6, 71) on CNTs. This type of trend is more obvious for functionalized CNTs compared with graphitized ones, which was attributed to the enhanced formation of water clusters or reduced H-bond formation when CNT carboxylic groups were ionized at elevated pHs (50). On the other hand, increased adsorption with increased pH was also observed and was attributed to enhanced EDA interactions (3, 37). The apparent pH influence on organic chemical adsorption depends on how the increase in attractive forces (e.g., EDA) counteracts the increase of repulsive forces (e.g., charge repulsion) and/or the decline of certain attractiveinteractions (e.g., H-bond formation and hydrophobic interaction). Valuable data may also be derived by comparing solution pH, the pKa of the organic chemical, and the pHpzc (point of zero charge) of CNTs. Low adsorption would be expected at pH > pKa and pHpzc because both adsorbent and adsorbate are negatively charged and electrostatic repulsion may be one of the dominant mechanisms. On the other hand, the organic chemical would show high adsorption under conditions with pHpzc > pH > pKa because of their electrostatic attraction with CNTs. The presence ofmetalions can bridge DOM and functional groups on CNTs, compress the double layer, neutralize negative charges of DOM, and thus weaken the repulsion between DOM molecules, and between DOM and CNTs (8, 70, 72). However, several questions remain unanswered. It is still unknown how the adsorption of metal ions alters the aggregation of CNT bundles, which consequently changes CNT adsorption with organic chemicals. Also, adsorption of mixed pollutants (including metals and organic chemicals) has not been widely investigated. The adsorption that is affected by the presence of another organic chemical could be understood from the competition between organic chemicals (73). But the presence of both metals and organic chemicals is a more complicated situation. Studies on wood charcoal adsorption properties have shown that coadsorption of copper ions decreased organic chemical sorption because of the formation of hydration shell (74). However, coadsorption of silver ions increased organic chemical sorption owing to the declined hydrophilicity of thelocal region around adsorbed silver ions, and thus reduced competitive sorption of water. Similar study on CNTs could provide useful information on organic chemical sorption mechanisms. Although significant effects of ionic strength on CNT adsorption characteristics has not been observed (43, 63), further research is needed, which will facilitate the prediction of organic chemical sorption on CNTs in a real environment. Dispersion of CNTs by Surfactants or DOM. Both surfactants (60, 75, 76) and DOM (7, 77, 78) have been reported to suspend CNTs significantly. Different dispersion mechanisms have been proposed, such as CNT solubilization inside surfactant columnar micelles (79), surfactant or DOM monolayer coatings on CNT surfaces (80), and “unzippering” of CNT bundles (81). Among all these mechanisms, surface coating of surfactants or DOM on CNTs is a key process. Although aromatic carbon content was observed to be proportional to DOM adsorption on CNTs, the amount of stable CNT suspension in aqueous phase did not follow a simple linear relationship with the adsorbed DOM concentration (72). The ability of a certain DOM to suspend CNTs is highly dependent on DOM properties. For example, hydrophobic DOM fraction may suspend CNTs more ef- ficiently than hydrophilic DOM fraction simply because of better contact of the hydrophobic fraction with the CNT surface (Figure 3). This speculation could be easily tested and may serve as a useful theory for effectively dispersing and suspending CNTs. Organic chemical-CNT interactions could be remarkably altered after CNT suspension. On one hand, the presence of surfactants or DOM could enhance the solubility of organic chemicals, and decrease their adsorption. On the other hand, surfactants or DOM can disperse CNT bundles and make more adsorption sites available for adsorbates, thus increasing the adsorption (82). The net impact depends on the balance of these two opposite factors as summarizedin Figure 4. Enhanced adsorption of phenanthrene (21), benzene, toluene, and n-undecane (82) on CNTs after being dispersed by surfactants was observed. The authors concluded that organic chemicals were able to interact more strongly with CNT surfaces in the presence of surfactants than without surfactants because of CNT dispersion by surfactants. Very few studies focused on CNT adsorption properties after being suspended by DOM. Although a humic acid (HA) had much lower sorption for phenanthrene, naphthalene, and Rnaphthol than CNTs, the HA-coated CNTs did not show distinct changes in sorption of these compounds relative to the original CNTs, probably due to the newly exposed sites VOL. 42, NO. 24, 2008 / ENVIRONMENTAL SCIENCE & TECHNOLOGY 9 9009 from enhanced dispersion as a result of the HA coating (83). Chen et al. (63) observed decreased adsorption of CNTs in the presence of DOM in the aqueous phase. However, no CNT suspension was involved in their study. Future studies need to consider the complexity of organic chemical behavior in an organic chemical-DOM-CNT three-phase system including fractionation of DOM upon adsorption on CNTs, nonideal interactions between organic chemicals and DOM, and disaggregation of CNT bundles. Dispersed CNTs are stable in various solvents, such as in water, toluene, and chloroform (84, 85). Thus dispersed CNTs and CNT-adsorbed organic chemicals can readily move in environmental media, which would subsequently facilitate the spread of various organic chemicals and thus increase their environmental risk, as expected from CNT’s high adsorption capacity. However, previous studies have rarely focused on CNT dispersion in relation to their adsorption with organic chemicals. One of the major reasons could be the difficulty in separating free dissolved organic chemicals from suspended CNTs. Because environmental risks of both CNTs and organic chemicals could be accurately assessed only if free dissolved organic chemicals could be separated and analyzed, proper separation methods need to be developed to study adsorption properties of suspended CNTs. Filtration (72, 78) and/or dialysis systems (86) may be applicable for separation purposes in future studies. Adsorption of Organic Chemicals by CNTs in Comparison with ACs CNTs, especially SWCNTs, have shown to be more efficient adsorbents than ACs and other adsorbents (Table SIII), with higher adsorption capacity (7, 13, 87), shorter equilibrium time (13, 50, 87), higher adsorption energy (88), and easier and more efficient regeneration (7, 8). As presented in Figure S5, SWCNTs generally have higher adsorption coefficients than ACs. For the above reasons, SWCNTs have the potential to be adsorbents for air purification and water treatment. Some studies reported low adsorption capacities of CNTs depending on their properties (27, 89). However, these authors speculate that an important part of CNT surfaces does not participate in adsorption processes (89), and adsorption capacity could be enhanced after proper treatment and processing, e.g., end-opening and functionalization. The unit price for ACs is currently much cheaper than that for SWCNTs, and thus they are widely used in air and water treatment. For the purpose of application in water treatment, numerous experiments have been conducted in column and pilot scales to evaluate ACs performance and operational cost (e.g., ref 90). However, such experiments are not available for CNTs. It should be emphasized that CNTs can be regenerated, maintaining high adsorption efficiency. Thus, they last longer than ACs do. Therefore, the FIGURE 3. CNT suspension as affected by DOM adsorption. HiDOM (solid line) stands for hydrophilic DOM fraction and HoDOM (dotted line) stands for hydrophobic fraction. Adsorption of HoDOM decreases with increased CNT oxygen content because of the decreased hydrophobicity of CNTs after oxidation. For the same reason, HiDOM follows the opposite trend (a). HoDOM suspends CNTs more efficiently than HiDOM (b) because a better contact between CNT and HoDOM is expected (c). FIGURE 4. Schematic diagram for dispersion of CNTs and their properties. The CNT aggregates can be dispersed after oxidation (a) or after coating with DOM or surfactants (c). Aggregate sizes (solid line) and zeta potentials (dotted line) decrease with increasing oxygen content (d) or DOM/surfactant concentration (e). For the adsorption of organic chemicals on CNTs dominated by hydrophobic effects, the oxidation of CNTs results in a decrease of adsorption because of the increased hydrophilicity of CNT surface. As they are further oxidized, the CNTs are dispersed and more adsorption sites are available, which may result in a slow rate of adsorption reduction (dashed line in panel d). However, for the adsorption of organic chemicals on CNTs dominated by H-bonds, adsorption would proportionally increase with oxygen content (dashed/dotted line in panel d). The adsorption of organic chemicals on CNTs may increase dramatically with the concentration of DOM or surfactant because of DOM fractionation and CNT dispersion (dashed and dashed/lines in panel e). Further increase of DOM or surfactants concentration in solution increases the solubility of organic chemicals strikingly, thus the apparent adsorption would decrease. 9010 9 ENVIRONMENTAL SCIENCE & TECHNOLOGY / VOL. 42, NO. 24, 2008 operational expense for CNTs in water treatment could be lower if they are properly regenerated. However, accurate estimate of these benefits and operational performance warrant investigations. Perspectives Various mechanisms may simultaneously control organic chemical adsorption on CNTs. Each adsorption mechanism may be affected differently by environmental conditions. For example, when H-bonds are the predominant mechanism, increased oxygen-containing functional groups on CNTs would increase the sorption. However, for sorption controlled by hydrophobic interactions, the increased functional groups would decrease the accessibility and affinity of CNTs for organic chemicals. Therefore, it is of great importance to obtain the relative contribution of different mechanisms to the overall sorption in the future. Comparisons between sorption results with or without a given mechanism may clarify the contribution and importance of individual mechanisms. For instance, sorption experiments conducted in aqueous phase complicate the discussion of sorption mechanisms because of the overwhelming hydrophobic effect. Analysis of sorption results obtained from sorption experiments in organic solvents with various polarities may help understand the contribution of hydrophobic effect. Nearly half of the current studies are theoretical simulations. Theoretical simulations often use vacuum conditions, not as they would behave in the real environment. Therefore, the results from the simulations may hardly be applicable in environmental conditions. More laboratory studies are required to systematically investigate the sorption of organic chemicals with different functional groups, hydrophobicity, and ability to donate or withdraw electrons (e.g., total Mulliken charge number). Also, very limited studies reported the effect of water chemistry on organic chemical sorption on CNTs. Extensive work is needed to examine the pH-, ionic strength-, and DOM-dependent sorption. Special concern should be directed to investigating CNT aggregation in different water chemistry conditions, and the resulting influence on sorption properties. As more and more CNTs enter the environment during CNT production, application, and disposal, environmental risk posed by CNTs will be controlled by their transport, exposure, and interaction with other pollutants. A better understanding of organic chemical-CNT interaction mechanisms and subsequent environmental behavior of both organic chemicals and CNTs will provide a fundamental basis for the prediction of CNT risk. In addition, being aware of CNT environmental risk helps to develop guidelines for safe design and application of CNTs. Numerous studies have recommended CNTs as alterative adsorbents in water treatment over ACs after batch adsorption experiments, but further work should investigate the performance and operational cost of CNTs in column and pilot scales. Acknowledgments This research was supported by the Massachusetts Water Resource Center (2007MA73B) and Massachusetts Agricultural Experiment Station (MA 90). We are also thankful to the four anonymous reviewers for their valuable comments to improve this manuscript. Supporting Information Available Three tables and five figures. These materials are available free of charge via the Internet at ACS Publications Home Page. Literature Cited (1) Nowack, B.; Bucheli, T. D. Occurrence, behavior and effects of nanoparticles in the environment. Environ. Pollut. 2007, 150, 5–22. (2) Mauter, M. S.; Elimelech, M. Environmental applications of carbon-based nanomaterials. Environ. Sci. Technol. 2008, 42, 5843–5859. (3) Helland, A.; Wick, P.; Koehler, A.; Schmid, K.; Som, C. Reviewing the environmental and human health knowledge base of carbon nanotubes. Environ. Health Persp. 2007, 115, 1125–1131. (4) Rao, G. P.; Lu, C.; Su, F. Sorption of divalent metal ions from aqueous solution by carbon nanotubes: A review. Sep. Purif. Technol. 2007, 58, 224–231. (5) Liu, G. H.; Wang, J. L.; Zhu, Y. F.; Zhang, X. R. Application of multiwalled carbon nanotubes as a solid-phase extraction sorbent for chlorobenzenes. Anal. Lett. 2004, 37, 3085–3104. (6) Pyrzynska, K.; Stafiej, A.; Biesaga, M. Sorption behavior of acidic herbicides on carbon nanotubes. Microchim. Acta 2007, 159, 293–298. (7) Su, F. S.; Lu, C. S. Adsorption kinetics, thermodynamics and desorption of natural dissolved organic matter by multiwalled carbon nanotubes.J. Environ. Sci. Health Part A 2007, 42, 1543– 1552. (8) Wang, S. G.; Liu, X. W.; Gong, W. X.; Nie, W.; Gao, B. Y.; Yue, Q. Y. Adsorption of fulvic acids from aqueous solutions by carbon nanotubes. J. Chem. Technol. Biotechnol. 2007, 82, 698–704. (9) Yang, K.; Xing, B. S. Desorption of polycyclic aromatic hydrocarbons from carbon nanomaterials in water. Environ. Pollut. 2007, 145, 529–537. (10) Ferguson, P. L.; Chandler, G. T.; Templeton, R. C.; Demarco, A.; Scrivens, W. A.; Englehart, B. A. Influence of sedimentamendment with single-walled carbon nanotubes and diesel soot on bioaccumulation of hydrophobic organic contaminants by benthic invertebrates. Environ. Sci. Technol. 2008, 42, 3879– 3885. (11) Agnihotri, S.; Rood, M. J.; Rostam-Abadi, M. Adsorption equilibrium of organic vapors on single-walled carbon nanotubes. Carbon 2005, 43, 2379–2388. (12) Brichka, S. Y.; Belyakova, L. A.; Prikhod’ko, G. P.; Roik, N. V. Surface structure and adsorption properties of multiwalled carbon nanotubes. Russ. Chem. Bull. 2006, 55, 1775–1779. (13) Lu, C. S.; Chung, Y. L.; Chang, K. F. Adsorption thermodynamic and kinetic studies of trihalomethanes on multiwalled carbon nanotubes. J. Hazard. Mater. 2006, 138, 304–310. (14) Hilding, J.; Grulke, E. A.; Sinnott, S. B.; Qian, D. L.; Andrews, R.; Jagtoyen, M. Sorption of butane on carbon multiwall nanotubes at room temperature. Langmuir 2001, 17, 7540–7544. (15) Yan, X. M.; Shi, B. Y.; Lu, J. J.; Feng, C. H.; Wang, D. S.; Tang, H. X. Adsorption and desorption of atrazine on carbon nanotubes. J. Colloid Interface Sci. 2008, 321, 30–38. (16) Yang, K.; Zhu, L. Z.; Xing, B. S. Adsorption of polycyclic aromatic hydrocarbons by carbon nanomaterials. Environ. Sci. Technol. 2006, 40, 1855–1861. (17) Shih, Y. H.; Li, M. S. Adsorption of selected volatile organic vapors on multiwall carbon nanotubes. J. Hazard. Mater. 2008, 154, 21–28. (18) Fagan, S. B.; Souza, A. G.; Lima, J. O. G.; Mendes, J.; Ferreira, O. P.; Mazali, I. O.; Alves, O. L.; Dresselhaus, M. S. 1,2- dichlorobenzene interacting with carbon nanotubes. Nano Lett. 2004, 4, 1285–1288. (19) Zhao, J. J.; Buldum, A.; Han, J.; Lu, J. P. Gas molecule adsorption in carbon nanotubes and nanotube bundles. Nanotechnology 2002, 13, 195–200. (20) Hirsch, A. Functionalization of single-walled carbon nanotubes. Angew. Chem., Int. Ed. 2002, 41, 1853–1859. (21) Gotovac, S.; Hattori, Y.; Noguchi, D.; Miyamoto, J.; Kanamaru, M.; Utsumi, S.; Kanoh, H.; Kaneko, K. Phenanthrene adsorption from solution on single wall carbon nanotubes. J. Phys. Chem. B 2006, 110, 16219–16224. (22) Gotovac, S.; Song, L.; Kanoh, H.; Kaneko, K. Assembly structure control of single wall carbon nanotubes with liquid phase naphthalene adsorption. Colloid Surf., A 2007, 300, 117–121. (23) Lee, J. W.; Kang, H. C.; Shim, W. G.; Kim, C.; Moon, H. Methane adsorption on multi-walled carbon nanotube at (303.15, 313.15, and 323.15) K. J. Chem. Eng. Data 2006, 51, 963–967. (24) Yudasaka, M.; Fan, J.; Miyawaki, J.; Iijima, S. Studies on the adsorption of organic materials inside thick carbon nanotubes. J. Phys. Chem. B 2005, 109, 8909–8913. (25) Agnihotri, S.; Kim, P.; Zheng, Y.; Mota, J. P. B.; Yang, L. C. Regioselective competitive adsorption of water and organic vapor mixtures on pristine single-walled carbon nanotube bundles. Langmuir 2008, 24, 5746–5754. (26) Vermisoglou, E. C.; Georgakilas, V.; Kouvelos, E.; Pilatos, G.; Viras, K.; Romanos, G.; Kanellopoulos, N. K. Sorption properties of modified single-walled carbon nanotubes. Microporous Mesoporous Mater. 2007, 99, 98–105. VOL. 42, NO. 24, 2008 / ENVIRONMENTAL SCIENCE & TECHNOLOGY 9 9011 (27) Bittner, E. W.; Smith, M. R.; Bockrath, B. C. Characterization of the surfaces of single-walled carbon nanotubes using alcohols and hydrocarbons: A pulse adsorption technique.Carbon 2003, 41, 1231–1239. (28) Chen, J.; Liu, H. Y.; Weimer, W. A.; Halls, M. D.; Waldeck, D. H.; Walker, G. C. Noncovalent engineering of carbon nanotube surfaces by rigid, functional conjugated polymers.J. Am. Chem. Soc. 2002, 124, 9034–9035. (29) Pan, B.; Lin, D. H.; Mashayekhi, H.; Xing, B. S. Adsorption and hysteresis of bisphenol A and 17R-ethinyl estradiol on carbon nanomaterials. Environ. Sci. Technol. 2008, 42, 5480–5485. (30) Wang, X. B.; Liu, Y. Q.; Qiu, W. F.; Zhu, D. B. Immobilization of tetra-tert-butylphthalocyanines on carbon nanotubes: A first step towards the development of new nanomaterials. J. Mater. Chem. 2002, 12, 1636–1639. (31) Chen, R. J.; Bangsaruntip, S.; Drouvalakis, K. A.; Kam, N. W. S.; Shim, M.; Li, Y. M.; Kim, W.; Utz, P. J.; Dai, H. J. Noncovalent functionalization of carbon nanotubes for highly specific electronic biosensors. Proc. Natl. Acad. Sci. U.S.A. 2003, 100, 4984–4989. (32) Shim, M.; Kam, N. W. S.; Chen, R. J.; Li, Y. M.; Dai, H. J. Functionalization of carbon nanotubes for biocompatibility and biomolecular recognition. Nano Lett. 2002, 2, 285–288. (33) Sander, M.; Lu, Y. F.; Pignatello, J. J. A thermodynamically based method to quantify true sorption hysteresis. J. Environ. Qual. 2005, 34, 1063–1072. (34) Balavoine, F.; Schultz, P.; Richard, C.; Mallouh, V.; Ebbesen, T. W.; Mioskowski, C. Helical crystallization of proteins on carbon nanotubes: A first step towards the development of new biosensors. Angew. Chem., Int. Ed. 1999, 38, 1912–1915. (35) Chen, W.; Duan, L.; Zhu, D. Q. Adsorption of polar and nonpolar organic chemicals to carbon nanotubes. Environ. Sci. Technol. 2007, 41, 8295–8300. (36) Lin, D. H.; Xing, B. S. Adsorption of phenolic compounds by carbon nanotubes: Role of aromaticity and substitution of hydroxyl groups. Environ. Sci. Technol. 2008, 42, 7254–7259. (37) Chen, W.; Duan, L.; Wang, L.; Zhu, D. Q. Adsorption of hydroxyland amino-substituted aromatics to carbon nanotubes.Environ. Sci. Technol. 2008, 42, 6862–6868. (38) Sumanasekera, G. U.; Pradhan, B. K.; Romero, H. E.; Adu, K. W.; Eklund, P. C. Giant thermopower effects from molecular physisorption on carbon nanotubes. Phys. Rev. Lett. 2002, 89, 1–4, 166801. (39) Cho, H. H.; Smith, B. A.; Wnuk, J. D.; Fairbrother, D. H.; Ball, W. P. Influence of surface oxides on the adsorption of naphthalene onto multiwalled carbon nanotubes. Environ. Sci. Technol. 2008, 42, 2899–2905. (40) Crespo, D.; Yang, R. T. Adsorption of organic vapors on singlewalled carbon nanotubes. Ind. Eng. Chem. Res. 2006, 45, 5524– 5530. (41) Mao, Z. G.; Sinnott, S. B. A computational study of molecular diffusion and dynamic flow through carbon nanotubes. J. Phys. Chem. B 2000, 104, 4618–4624. (42) Mao, Z. G.; Sinnott, S. B. Separation of organic molecular mixturesin carbon nanotubes and bundles:Molecular dynamics simulations. J. Phys. Chem. B 2001, 105, 6916–6924. (43) Gotovac, S.; Yang, C. M.; Hattori, Y.; Takahashi, K.; Kanoh, H.; Kaneko, K. Adsorption of polyaromatic hydrocarbons on single wall carbon nanotubes of different functionalities and diameters. J. Colloid Interface Sci. 2007, 314, 18–24. (44) Tournus, F.; Charlier, J. C. Ab initio study of benzene adsorption on carbon nanotubes. Phys. Rev. B 2005, 71, 1–8, 165421. (45) Hilding, J. M.; Grulke, E. A. Heat of adsorption of butane on multiwalled carbon nanotubes. J. Phys. Chem. B 2004, 108, 13688–13695. (46) Davis, J. J.; Green, M. L. H.; Hill, H. A. O.; Leung, Y. C.; Sadler, P. J.; Sloan, J.; Xavier, A. V.; Tsang, S. C. The immobilisation of proteins in carbon nanotubes.Inorg. Chim. Acta 1998, 272, 261– 266. (47) Onyestyak, G.; Otvos, Z.; Valyon, J.; Kiricsi, I.; Rees, L. V. C. Acetylene sorption dynamics in carbon nanotubes. Helv. Chim. Acta 2004, 87, 1508–1514. (48) Datsyuka, V.; Kalyva, M.; Papagelis, K.; Parthenios, J.; Tasisb, D.; Siokoua, A.; Kallitsisa, I.; Galiotisa, C. Chemical oxidation of multiwalled carbon nanotubes. Carbon 2008, 46, 833–840. (49) Liu, C. H.; Li, J. J.; Zhang, H. L.; Li, B. R.; Guo, Y. Structure dependent interaction between organic dyes and carbon nanotubes. Colloid Surf., A 2008, 313, 9–12. (50) Peng, X. J.; Li, Y. H.; Luan, Z. K.; Di, Z. C.; Wang, H. Y.; Tian, B. H.; Jia, Z. P. Adsorption of 1,2-dichlorobenzene from water to carbon nanotubes. Chem. Phys. Lett. 2003, 376, 154–158. (51) Ago, H.; Kugler, T.; Cacialli, F.; Salaneck, W. R.; Shaffer, M. S. P.; Windle, A. H.; Friend, R. H. Work functions and surface functional groups of multiwall carbon nanotubes.J. Phys. Chem. B 1999, 103, 8116–8121. (52) Piao, L. Y.; Liu, Q. R.; Li, Y. D.; Wang, C. Adsorption of L-phenylalanine on single-walled carbon nanotubes. J. Phys. Chem. C 2008, 112, 2857–2863. (53) Liao, Q.; Sun, J.; Gao, L. The adsorption of resorcinol from water using multi-walled carbon nanotubes. Colloid Surf., A 2008, 312, 160–165. (54) Ahnert, F.; Arafat, H. A.; Pinto, N. G. A study of the influence of hydrophobicity of activated carbon on the adsorption equilibrium of aromatics in non-aqueous media. Adsorption 2003, 9, 311–319. (55) Zhu, D. Q.; Kwon, S.; Pignatello, J. J. Adsorption of single-ring organic compounds to wood charcoals prepared under different thermochemical conditions. Environ. Sci. Technol. 2005, 39, 3990–3998. (56) Ania, C. O.; Cabal, B.; Pevida, C.; Arenillas, A.; Parra, J. B.; Rubiera, F.; Pis, J. J. Effects of activated carbon properties on the adsorption of naphthalene from aqueous solutions. Appl. Surf. Sci. 2007, 253, 5741–5746. (57) Hickey, J. P.; Passinoreader, D. R. Linear solvation energy relationships - rules of thumb for estimation of variable values. Environ. Sci. Technol. 1991, 25, 1753–1760. (58) Gotovac, S.; Honda, H.; Hattori, Y.; Takahashi, K.; Kanoh, H.; Kaneko, K. Effect of nanoscale curvature of single-walled carbon nanotubes on adsorption of polycyclic aromatic hydrocarbons. Nano Lett. 2007, 7, 583–587. (59) Karajanagi, S. S.; Vertegel, A. A.; Kane, R. S.; Dordick, J. S. Structure and function of enzymes adsorbed onto single-walled carbon nanotubes. Langmuir 2004, 20, 11594–11599. (60) Richard, C.; Balavoine, F.; Schultz, P.; Ebbesen, T. W.; Mioskowski, C. Supramolecular self-assembly of lipid derivatives on carbon nanotubes. Science 2003, 300, 775–778. (61) Gurevitch, I.; Srebnik, S. Conformational behavior of polymers adsorbed on nanotubes. J. Chem. Phys. 2008, 128, 1–8, 144901. (62) Zhou, G.; Duan, W. H.; Gu, B. L. First-principles study on morphology and mechanical properties of single-walled carbon nanotube. Chem. Phys. Lett. 2001, 333, 344–349. (63) Chen, J.; Chen, W.; Zhu, D. Q. Adsorption of nonionic aromatic compounds to single-walled carbon nanotubes: Effects of aqueous solution chemistry. Environ. Sci. Technol. 2008, 42, 7225–7230. (64) Star, A.; Stoddart, J. F.; Steuerman, D.; Diehl, M.; Boukai, A.; Wong, E. W.; Yang, X.; Chung, S. W.; Choi, H.; Heath, J. R. Preparation and properties of polymer-wrapped single-walled carbon nanotubes. Angew. Chem., Int. Ed. 2001, 40, 1721–1725. (65) Zhao, J. J.; Lu, J. P.; Han, J.; Yang, C. K. Noncovalent functionalization of carbon nanotubes by aromatic organic molecules. Appl. Phys. Lett. 2003, 82, 3746–3748. (66) Woods, L.M.; Badescu, S. C.; Reinecke, T. L. Adsorption of simple benzene derivatives on carbon nanotubes. Phys. Rev. B 2007, 75, 1–9, 155415. (67) Lu, C.; Su, F.; Hu, S. Surface modification of carbon nanotubes for enhancing BTEX adsorption from aqueous solutions. Appl. Surf. Sci. 2008, 254, 7035–7041. (68) Liao, Q.; Sun, J.; Gao, L. Adsorption of chlorophenols by multiwalled carbon nanotubes treated with HNO3 and NH3. Carbon 2008, 46, 553–555. (69) Sone, H.; Fugetsu, B.; Tsukada, T.; Endo, M. Affinity-based elimination of aromatic VOCs by highly crystalline multi-walled carbon nanotubes. Talanta 2008, 74, 1265–1270. (70) Lu, C. Y.; Su, F. S. Adsorption of natural organicmatter by carbon nanotubes. Sep. Purif. Technol. 2007, 58, 113–121. (71) Biesaga, M.; Pyrzynska, K. The evaluation of carbon nanotubes as a sorbent for dicamba herbicide. J. Sep. Sci. 2006, 29, 2241– 2244. (72) Hyung, H.; Kim, J. H. Natural organic matter (NOM) adsorption tomulti-walled carbon nanotubes: Effect of NOM characteristics and water quality parameters. Environ. Sci. Technol. 2008, 42, 4416–4421. (73) Yang, K.; Wang, X. L.; Zhu, L. Z.; Xing, B. S. Competitive sorption of pyrene, phenanthrene, and naphthalene on multiwalled carbon nanotubes. Environ. Sci. Technol. 2006, 40, 5804–5810. (74) Chen, J. Y.; Zhu, D. Q.; Sun, C. Effect of heavy metals on the sorption of hydrophobic organic compounds to wood charcoal. Environ. Sci. Technol. 2007, 41, 2536–2541. (75) Ham, H. T.; Choi, Y. S.; Chung, I. J. An explanation of dispersion states of single-walled carbon nanotubes in solvents and aqueous surfactant solutions using solubility parameters. J. Colloid Interface Sci. 2005, 286, 216–223. 9012 9 ENVIRONMENTAL SCIENCE & TECHNOLOGY / VOL. 42, NO. 24, 2008 (76) Vaisman, L.; Wagner, H. D.; Marom, G. The role of surfactants in dispersion of carbon nanotubes. Adv. Colloid Interface Sci. 2006, 128, 37–46. (77) Hyung, H.; Fortner, J. D.; Hughes, J. B.; Kim, J. H. Natural organic matter stabilizes carbon nanotubes in the aqueous phase. Environ. Sci. Technol. 2007, 41, 179–184. (78) Lin, D. H.; Xing, B. S. Tannic acid adsorption and its role for stabilizing carbon nanotube suspensions. Environ. Sci. Technol. 2008, 42, 5917–5923. (79) O’Connell, M. J.; Bachilo, S. M.; Huffman, C. B.; Moore, V. C.; Strano, M. S.; Haroz, E. H.; Rialon, K. L.; Boul, P. J.; Noon, W. H.; Kittrell, C.; Ma, J. P.; Hauge, R. H.; Weisman, R. B.; Smalley, R. E. Band gap fluorescence from individual single-walled carbon nanotubes. Science 2002, 297, 593–596. (80) Matarredona, O.; Rhoads, H.; Li, Z. R.; Harwell, J. H.; Balzano, L.; Resasco, D. E. Dispersion of single-walled carbon nanotubes in aqueous solutions of the anionic surfactant NaDDBS.J. Phys. Chem. B 2003, 107, 13357–13367. (81) Bandyopadhyaya, R.; Nativ-Roth, E.; Regev, O.; YerushalmiRozen, R. Stabilization of individual carbon nanotubes in aqueous solutions. Nano Lett. 2002, 2, 25–28. (82) Carrillo-Carrion, C.; Lucena, R.; Cardenas, S.; Valcarcel, M. Surfactant-coated carbon nanotubes as pseudophases in liquidliquid extraction. Analyst 2007, 132, 551–559. (83) Wang, X. L.; Lu, J. L.; Xing, B. S. Sorption of organic contaminants by carbon nanotubes: Influence of adsorbed organic matter. Environ. Sci. Technol. 2008, 42, 3207–3212. (84) Petrov, P.; Stassin, F.; Pagnoulle, C.; Jerome, R. Noncovalent functionalization of multi-walled carbon nanotubes by pyrene containing polymers. Chem. Commun. 2003, 2904–2905. (85) Lou, X. D.; Daussin, R.; Cuenot, S.; Duwez, A. S.; Pagnoulle, C.; Detrembleur, C.; Bailly, C.; Jerome, R. Synthesis of pyrenecontaining polymers and noncovalent sidewall functionalization of multiwalled carbon nanotubes.Chem. Mater. 2004, 16, 4005– 4011. (86) Pan, B.; Ghosh, S.; Xing, B. S. Nonideal binding between dissolved humic acids and polyaromatic hydrocarbons. Environ. Sci. Technol. 2007, 41, 6472–6478. (87) Lu, C. S.; Chung, Y. L.; Chang, K. F. Adsorption of trihalomethanes from water with carbon nanotubes. Water Res. 2005, 39, 1183– 1189. (88) Long, R. Q.; Yang, R. T. Carbon nanotubes as superior sorbent for dioxin removal. J. Am. Chem. Soc. 2001, 123, 2058–2059. (89) Diaz, E.; Ordonez, S.; Vega, A. Adsorption of volatile organic compounds onto carbon nanotubes, carbon nanofibers, and high-surface-area graphites. J. Colloid Interface Sci. 2007, 305, 7–16. (90) Crittenden, J. C.; Reddy, P. S.; Arora, H.; Trynoski, J.; Hand, D.W.; Perram, D. L.; Summers, R. S. Predicting GAC performance with rapid small-scale column tests. J. Am. Water Works Assoc. 1991, 83, 77–87. ES801777N VOL. 42, NO. 24, 2008 / ENVIRONMENTAL SCIENCE & TECHNOLOGY 9 9013 The author has requested enhancement of the downloaded file. All in-text references underlined in blue are linked to publications on ResearchGate.
What is the recent advancement in mass spectrometry?
New mass spectrometry (MS) methods, collectively known as data independent analysis and hyper reaction monitoring, have recently emerged. These methods hold promises to address the shortcomings of data-dependent analysis and selected reaction monitoring (SRM) employed in shotgun and targeted proteomics, respectively.Proteomic strategies can be divided into two approaches according to their purpose (Fig. 1). One is peptide mass fingerprinting (PMF) by MALDI-MS, which is used for detailed protein identification in a high-throughput manner (Lam, Li, Lo, Guggenheim, & To, 2007). In this method, it is important to isolate proteins as much as possible using two-dimensional electrophoresis (2D-PAGE). The other is shotgun proteomics, which is used for global peptide identification by hybrid MS (precursor ion selection, MS1, and then product ion detection, MS2). In the shotgun method, it is necessary to first digest the protein mixture, and then accurately separate the large number of generated peptides using high-performance LC (Stephanowitz, Lange, Lang, Freund, & Krause, 2012). From the viewpoint of protein separation, 2D-PAGE is one of the best protein separation protocols. However, this method has issues such as a complicated procedure and poor reproducibility. Immobilized pH gradient gels and automated 2D-PAGE systems have been developed, but these still have not lead to solutions for intrinsic recovery. Currently, the first choice for proteomic approaches is usually LC-ESI-MS, because of improvements in nanoliter-flow LC, column resins, probe performance, automated MS and MS/MS analysis, and the basic potential for MS and its peripheral devices (Howells et al., 2005, Iwasaki et al., 2010, Sun et al., 2014). In addition, MS is a platform that analyzes molecules after they are ionized into the gas phase. Therefore, the molecules must be ionized in some way, and the ionization technologies, fast atom bombardment (FAB) (Morris, Panico, Karplus, Lloyd, & Riniker, 1982), electron ionization (EI), chemical ionization (CI) (Oka et al., 1991), and specific chemical modification are available (Calderon-Celis, Encinar, & Sanz-Medel, 2017). MALDI and ESI are widely used, especially for biomolecules (Hommerson, Khan, de Jong, & Somsen, 2011). These ionization reactions are called “soft ionization” methods. Since the precursor ions of the biomolecule are usually not cleaved, structure-derived product ions from the biomolecule are easily detected (Standing, 2003).Fig. 1Fig. 1. Two proteomic approaches by shotgun LC-MS/MS analysis and 2D-PAGE/PMF.Proteins are extracted correctly from biological samples. 1) Proteins are first digested by trypsin protease after denaturation and reductive alkylation. And generated peptides are separated by reverse-phase HPLC, and directly induced into ESI interface. MS and MS/MS spectra are obtained by data-dependent acquisition. 2) Proteins are denatured in reductive chaotropic solution with non-ionic detergent, and separated by isoelectric point and molecular weight. Each protein spots are digested by trypsin after reductive alkylation. PMF spectra are obtained after purification and mixing with ionization matrix. Protein identification is performed by proteome database server by using processed MS/MS and PMF spectra for the determination of post-translational modification or molecular annotation.Additionally, the amount of detected ions can also be measured. Considering the feature of global MS analysis, a dataset of containing ion amounts with molecular information has great significance when studying a biological system. One popular area that uses proteomics is the development of clinical biomarkers (Jingushi et al., 2017, Nirmalan et al., 2011). Biomarker signatures are defined as an index that can be measured in the body fluids and tissues and used to evaluate objectively the pharmacological responses during normal biological, pathological processes, and practical treatments (Rifai et al., 2006, Vasan, 2006). Moreover, these biomarkers are verified as indicators for the prediction of disease, prognosis, and individual differences. Biomarker rules are roughly classified into three categories: reference limits that statistically derive cut-off points based on the reference samples, discrimination limits that separate patients with a disease from those without it, and threshold follow-ups that identify the level of disease. As a therapeutic index, the blood level monitoring of therapeutic drugs can be visualized, and the treatment responses can be quantified to support practical decisions. Biomarkers can be distinctly subdivided in accordance with their purpose. Starting from broad categories like diagnostic markers or companion diagnosis, biomarkers suitable for a situation have more clinical significance, such as prognostic, predictive, surrogate, monitoring, toxicity, pharmacodynamics (PD), autoantigen, and stratification markers (Poste et al., 2012). The strategies for biomarker discovery differ largely according to the target for the therapeutic or diagnostic phase. A biological system exerts its functions by controlling the expression of many molecules in response to constantly changing phenomena. Even though phenotypic surfaces are silently homeostatic, systems are predicted to be based on various controls in order to maintain the biological process (Kurachi et al., 2009). Taking this situation into consideration, a disease profile and mechanism can be elucidated in detail using an identical biological sample set from the initiation of disease with inflammatory symptoms, pathogenic events to regenerative recovery. Integrated proteomic strategies with protein expression and time-dependent profile changes leads to the discovery of more in-depth biomarkers (Shimada et al., 2010). Quantitative proteomic views and approaches are indispensable for analyzing these systems biology.In this review, we discuss the principles and applications of MS in the context of the trend of quantitative proteomics based on biomolecular-specific structures, and the analytical advances in MS and their contributions to the life science, medical, and pharmaceutical fields. We also describe our recent advances in MS-based bioanalysis and how they have contributed to the remarkable development of antibody drugs and their therapeutic applications.2. Structure-indicated quantitation by mass spectrometry2.1. MS/MS fragmentation, accuracy, and resolution for peptide identificationCurrently, in the proteomic field with MS, accurate protein identification is generally performed using a dataset of precursor and product ions generated by collision-induced dissociation (CID) in hybrid MS function. Peptide fragmentation patterns depend on several factors such as amino acid sequence, covalent potential of amide or other covalent bonds, and ion valence. In a peptide bond dissociation reaction, product ions derived from amide bonds are preferentially cleaved and detected because of the larger dissociation constant of the amide compared to that of residues to α‑carbon, and high probability of charge localization on the amide by weak conjugation formation. Consequently, the detection probability derived from the b- and y-ion series increases. These ion series are most commonly used for structure assignment because they are reflected in peptide-specific amino acid sequences (Guthals & Bandeira, 2012).The atomic content of peptides includes a naturally constant ratio of stable isotopes. For the exact mass, the lowest m/z peak in no isotope distribution, the monoisotopic mass, is selected (Valkenborg, Jansen, & Burzykowski, 2008). The selection of the monoisotopic peak should be carried out as accurately as possible. Peak processing errors lead to a mass difference of 1 Da or more. Proteomic analysis involves a huge number of peptides as analytical targets, and several precursor ions are frequently detected in the near m/z. Therefore, peak processing errors definitely prevent protein identification (DeFelice et al., 2017). On the other hand, high-resolution MS greatly aids in identification and structure assignment. Isoleucine and leucine are isobaric and cannot be separated, but it is possible to distinguish between glutamate and leucine (mass δ = 0.036 Da). Moreover, neutral loss ions of deamidation (δ = 17 Da) and dehydration (δ = 18 Da) can be also reliably separated. As proteomic strategies are designed to be more accurate, high-resolution MS becomes more essential.2.2. Protein identification by mass spectrometry data processingProteomic analysis is assisted seamlessly by excellent MS data processing algorithms. The exact monoisotopic peaks of peptide ions from the protein digestion procedure are acquired, and a peak list of the combination of the precursor and generated product ion information with ion valence is produced. Then, this peak list is entered into the database analysis server, which returns with the ion scores of each peptide. The ion scores of the peptides are calculated using an appropriate scoring algorithm with a theoretical peptide fragment dataset generated from submitted sequence databases. The closest match or matches for a peptide can be identified using the identification criteria of the reliability scores. Currently, Mascot (Matrix Science, UK) (Eng et al., 1994, Pappin et al., 1993), ProteinProspector (UCSF, US) (Chalkley et al., 2005), ProteinPilot (Sciex, US), Proteome Discoverer (Thermo Fisher, US), ProteinScape (Bruker, DE), and Expressionist (Genedata, Switzerland) (Ueda et al., 2011) are the main active well-known proteome servers, and are indispensable engines for large-scale proteomics. For the functional annotation and statistical analysis of identified proteins, Gene Ontology (Gene Ontology Resource) (Camon et al., 2004, Zeeberg et al., 2003) and DABID Bioinformatics (http://david.ncifcrf.gov/) (Huang da, Sherman, & Lempicki, 2009) provide web-accessible engines to visualize biological investigation from several omics dataset. Furthermore, ProteinAtras (http://www.proteinatlas.org/) (Uhlen et al., 2010, Uhlen et al., 2015) and ExSPASy (https://www.expasy.org/) (Artimo et al., 2012) were built as unified protein knowledgebases.The process of data analysis and annotation of large scale dataset from MS is extremely significant for the proteomics-based sciences, which is why, MS is also referred to as an information science.2.3. Comprehensive quantitation for overall systems biologyA quantitative proteomic dataset with high reproducibility provides valuable biological impacts. Specifically, when elucidating a biological landscape as a system, unified strategies combined with multi-omics technologies are necessary. To quantitate the proteins comprehensively, individual ion peaks derived from identified proteins are determined. For more effective and successive quantitation, a targeted quantitation method is currently carried out. The hybrid triple quadrupole (TQ) MS function of selecting a precursor ion from tryptic peptides by the 1st quadrupole (Q1), CID reaction with Argon or a rare gas in the 2nd quadrupole (Q2), and selection of a structure-specific product ion by the 3rd quadrupole (Q3) is optimal for the accurate and successive quantitation of the proteome. The combination of the precursor (Q1) and product ion (Q3) m/z is named the transition of multiple reaction monitoring (MRM) or selected reaction monitoring (SRM). Global quantitative analysis becomes possible by switching this transition at high speed. However, the transition switching speed has an upper limit, and there are some cases in which the MRM method alone cannot perform a satisfactory quantitation of large-scale proteomics due to the prevalence of high-performance LC. Additionally, in recent years, the MRM method has been improved to the parallel reaction monitoring (PRM) (Kreimer et al., 2017). In PRM, a specific product ion is not selected by Q3, but all fragment ions are detected and quantitated after data acquisition. Compared with MRM, the features of PRM encompass a wider range of conditions for quantitative use. Moreover, PRM can be used to clarify the quantitative structure indexes and transition interference from a biological matrix. The database resources PeptideAtras (PeptideAtlas) (Desiere et al., 2006), Skyline (http://skyline.ms/project/home/software/Skyline/begin.view) (MacLean et al., 2010), MaxQuant (http://www.biochem.mpg.de/5111795/maxquant) (Cox & Mann, 2008), and iMPAQT (http://www.bioreg.kyushu-u.ac.jp/saibou/index_en.html) (Matsumoto et al., 2017) are currently being advanced and contributed to the accurate quantitative proteomics.Matsumoto M and Nakayama K et al. have performed excellent study to elucidate the landscape of metabolic systems by identifying and quantifying the global metabolic enzymes on the pathway under the assumption that the expression levels of proteins are the rate-limiting factors in metabolic regulation (Matsumoto et al., 2017). In order to quantitate an individual protein within the genome-wide proteome, they first developed the proteotypic peptide (PTP) (Michalski et al., 2011, Razavi et al., 2012, Selevsek et al., 2015), which can be used to perform the specific quantitation of an individual protein. PTPs are selected from among the tryptic peptides of recombinant human proteins from full-length human cDNA libraries (Goshima et al., 2008). By using actual MS data for the selection of PTPs, optimal PTP selection has become possible through the overall consideration of the efficiency of digestion, peptide release, and ionization. Moreover, the copy number of expressed protein can be determined using the stable isotope-labeled (SIL) PTP as an internal standard (Howden et al., 2013, Ong et al., 2002). Using this strategy, the quantitation of a low-abundance proteome is possible with a wide dynamic range.3. Bioanalytical technologies for protein biopharmaceuticals3.1. Recent advancements in antibody drugs and the necessity of clinical indicators for drug efficacySince the 1980s, specific molecules and pathways related to individual diseases have been identified, and the research to elucidate the molecular mechanism has advanced dramatically. These achievements accelerated the development of molecular target drugs that inhibit a specific molecule and signaling pathway. The approval of anti-CD20 Rituximab for lymphoma (Maloney et al., 1997), anti-ErbB2 Trastuzumab for breast cancer (Baselga, Norton, Albanell, Kim, & Mendelsohn, 1998), and anti-TNFα Infliximab for rheumatoid arthritis (Targan et al., 1997) contributed to the acceleration of the development of antibody drugs in the late 1990s. Recently, antibody drugs against CD antigens, receptors, growth factors, cytokines, immune checkpoints, infectious factors, and amyloid beta have been developed, and about more 60 drugs have been approved as molecular targeted antibody drugs (Mullard, 2017a, Mullard, 2017b). Furthermore, more than 500 items and their biosimilars have been developed, and further improvements are expected. In fact, the combined administration of low-molecular-weight and antibody drugs against a specific disease has become a standard treatment (Avallone et al., 2016). On the other hand, drug efficacy, toxicity, and side effects are correlated with individual differences (Ito, Kondo, Tada, & Kitano, 2015). Thus, treatment has evolved into individualized and optimized medicine in which a patient group that is expected to receive medicinal treatment is selected scientifically before the practical treatment. In the realization of individualized medicine, additional information such as on the expression level of the target molecules, genetic background, indicators of drug efficacy, toxicity biomarkers, immunoreactivity, and metabolism are extremely important.The drug efficacy of small-molecular target drugs typically has a narrow therapeutic window, and blood concentration is one of a key surrogate efficacy index for the appropriate dosage for an individual. Currently, for the anti-cancer agents methotrexate (Przybylski, Preiss, Dennebaum, & Fischer, 1982) and imatinib (Beumer, 2013, Hamada et al., 2003), and pharmaceuticals such as immunosuppressive agents cyclosporine and tacrolimus, it is possible to perform effective treatment maintenance by carrying out therapeutic drug monitoring (Wong, 2001). On the other hand, the metabolic pathway and drug efficacy index of antibody drugs remain unclear, and as a result, the dosage level is not determined enough. However, a positive correlation between the blood concentration and survival was reported about in recent clinical trials of Trastuzumab (Kang et al., 2014) and Ramucirumab (Tabernero et al., 2017) for progressive gastric cancer. Moreover, in a clinical study of Infliximab and Adalimumab against rheumatoid arthritis (Mulleman et al., 2009, Pouw et al., 2015), the blood concentration and inflammation level were inversely correlated. Furthermore, the overproduction of anti-drug antibodies as one of the causes has been addressed (G. P. Eng et al., 2015, Marinari et al., 2014). In 2013, cancer immunotherapy was selected as the breakthrough of the year (Couzin-Frankel, 2013, Ishida et al., 1992): by inhibiting the binding of PD-1/PD-L1, it activates cytotoxic T cells, and lead to the attack to cancer cells that originally escaped from detection by immune system. The antibody drug will most likely be applied to many cancers as the notable treatments of the fourth option, immunotherapy, following surgery, chemotherapy, and radiation. In addition, for the novel therapeutic strategy of controlling the delicate balance of the immune system, the phenotypic response, which had not been anticipated, has been confirmed, and the development of an index that can be used to accurately evaluate efficacy, toxicity, and other factors, has become an urgent issue (Davies and Duffield, 2017, Gao et al., 2012). In the near future, the development of therapeutic methods incorporating scientific indicators for treatment using antibody drugs is required.3.2. Monitoring of protein biopharmaceuticals using current proteomic approachesThe ligand binding assay (LBA) is generally used as a bioanalytical method for monitoring antibody drugs. The LBA method, consists of a specific antibody against an antigen, the purification and concentration of the antigen from the biological matrix, and then a sandwich immunoassay using the detected antibody. Although this detection method mainly involves colorimetric and chemiluminescence produced by an enzymatic reaction such as horseradish peroxidase, an analysis with higher selectivity is possible using MS. This method is called hybrid LBA/LC-MS or the mass spectrometric immunoassay (Trenchevska, Nelson, & Nedelkov, 2016).In bioanalysis using hybrid LBA/LC-MS, in addition to the evaluation of selectivity and the recovery rate from the matrix used in the usual validation test, it is also necessary to validate the affinity capture efficiency of the enzyme and the digestion efficiency. Moreover, in the LBA, it is very important to assess the lot-to-lot variability of the capturing antibody or ligand. In consideration of these points, to establish the internal standard material, the SIL of authentic samples of the full length of the antibody to be analyzed is an ideal procedure (Ismaiel, Mylott, & Jenkins, 2017). Furthermore, the accelerated production of endogenous antibodies against antibody drugs is considered to interfere with precise measurements (Bader et al., 2017). In particular, it is possible to directly affect the affinity capture efficiency. To address this issue, the whole digest samples from the serum are used, and the signature peptide to be selected from the LC-MS is quantified based on the technology of conventional shotgun proteomics. With this technique, it is possible to perform a bioanalysis dependent only on the selectivity potential of high performance MS.3.3. Novel approach of Fab-selective proteolysis nSMOLThe method of LBA or hybrid LBA/LC-MS requires an affinity antibody against each antibody drug and it has become difficult to maintain the analytical the quality in the recent clinical advancement. We confirmed that the influence on the MS quantified value and its behavior differ depending on the type of idiotypic antibody against each antibody drug (Iwamoto, Hamada, & Shimada, 2017). This suggests that it is difficult to prepare an appropriate affinity antibody clones or specific ligands for hybrid LBA/LC-MS, and confirmation of the actual value is essential for the individual clinical difference. And by the whole digest approaches, it is difficult to maintain the ESI interface clearance because complex biological samples have a large excess of peptide analytes (Liu et al., 2012). In the protein bioanalysis using MS, there are several principle issues to overcome, such as the problem of separation ability and carryover in the chromatograph portion, the ion suppression effect and desolvation efficiency of the target molecule due to coexisting ions in the MS, and the handling of multiple sample processing. Accordingly, the development of bioanalytical methods, which make use of the features of MS such as high selectivity, completeness, and continuity, is needed involving the overall optimization of sample processing, biochemistry, structure, enzyme reaction and release efficiency, material sciences, column separation, ionization efficiency, and throughput ability, to contribute to clinical chemistry and pharmaceutical science.We proposed a novel analytical method based on the structure specificity of an antibody independent of a variety of monoclonal antibodies, and this basic technology has been widely applicable to the development and clinical use of antibody drugs. Immunoglobulin G (IgG) is the main antibody drug that has been analyzed. IgG has a 15 nm molecular diameter and is tetramer protein that has two heavy and light chains. IgG is the second major proteome in blood protein content. Since the structural features representing the antigen specificity of antibodies are determined by amino acid substitution through somatic hypermutation on the variable fragment Fv (Novotny and Bruccoleri, 1987, Wu and Kabat, 1970), universal antibody analysis becomes possible by selectively quantitating the complementarity-determining regions (CDRs). Based on these considerations, we developed a novel method, nano-surface and molecular-orientation limited (nSMOL) proteolysis (Iwamoto et al., 2014), for the bioanalysis of antibody drugs (Fig. 2). The nSMOL method consists of orientating the fragment antigen-binding (Fab) towards the reaction solution side by binding IgG in a porous body with a pore diameter of 100 nm via the constant region Fc, and immobilizing the protease on the surface of the nanoparticles with a diameter of 200 nm, resulting in physicochemically limiting the access of the protease to the immobilizing IgG substrate. In this solid-solid reaction, Fab-selective proteolysis becomes possible, and consequently, it is possible to greatly reduce the complexity of the peptide to be analyzed by holding the structural specificity of IgG and eliminating the contamination of the protease. The peptides that include CDRs are candidate signature peptides for antibody drug determination and quantitation, and it is possible to provide high sensitivity, high selectivity, and universal use of analytical technology with appropriately selecting MRM transitions that have antibody structure specificity.Fig. 2 .Fig. 2. Concept representation of nSMOL proteolysis.IgG molecules are collected in the porous resin with 100 nm diameter via fragment, crystallizable (Fc), so that fragment, antigen-binding (Fab) is oriented towards reaction solution. Modified trypsin (shown in yellow picture) is immobilized on the surface of nanoparticle with 200 nm diameter. In this solid-solid reaction, trypsin access to IgG substrate is physically limited, and Fab-selective proteolysis successfully continues. Consequently, it is possible to greatly reduce the complexity of the peptide analyte by holding the structural specificity of IgG on CDR and eliminating contamination of protease, and providing the high-sensitivity and high-selectivity quantitation of universal antibody bioanalysis. (For interpretation of the references to colour in this figure legend, the reader is referred to the web version of this article.)Even the good antibody with high-specificity and high-affinity to the ligand, bioanalytical validation using the common LBA-related methods surely require the one antibody substrate for one ligand. This one-to-one approach is not only difficult to apply into the broad and rapid clinical studies, but also the cost for preparation and quality control of biological components become a critical issue. However, using Fab-selective reaction nSMOL coupled with mass-based resolution LC-MS/MS, bioanalytical validation for many monoclonal antibodies become possible on the identical platform. And the nSMOL can be configured the condition setting independent of animal matrices. This feature means that comparative data analysis may be addressed from the initial stage of antibody development in animal model to the first-in-human and late-phase clinical trials, and the contribution to antibody drug discovery. Moreover, this strategy can accelerate the cross-sectional trials on rare diseases, and drug-drug interaction assessment for the new combination therapy.The analytical reliability of antibody drug analysis using nSMOL is based on the “Enforcement Regulations of the Law Concerning the Quality, Effectiveness and Security of Securing Drugs, Medical Equipment, etc.” and “Guideline on Bioanalytical Method Validation in Pharmaceutical Development”. In order to verify that nSMOL proteolysis accompanied by TQ-LC-MS analysis meets the criteria of these guideline, the authors have already carried out and reported on the full validation analysis against various antibody drugs (Trastuzumab (Iwamoto, Yamane, Umino, Hamada, & Shimada, 2015), Bevacizumab (Iwamoto, Umino, et al., 2016), Cetuximab (Iwamoto, Takanashi, et al., 2016), Rituximab (Iwamoto, Takanashi, Hamada, & Shimada, 2016b), Nivolumab (Iwamoto, Shimada, Terakado, and Hamada, 2016), Brentuximab vedotin (Iwamoto, Takanashi, Hamada, & Shimada, 2016a), and Trastuzumab emtansine (Iwamoto, Shimomura, Tamura, Hamada, & Shimada, 2017)) using nSMOL. Moreover, since the nSMOL reacts to whole IgG molecules in the blood in principle, multiplex quantitation is possible for the application of combination cancer therapy and therapeutic drug monitoring for inflammatory immune diseases (Iwamoto et al., 2016d, Messenheimer et al., 2017, Steenholdt et al., 2014). nSMOL results have demonstrated that blood concentrations in the range of about 0.1 to 300 μg/mL fulfill all validation criteria for antibody drugs, and it has both analytical reliability and multipurpose universal use (Table 1). This quantifiable range will fulfill without the requirements of any additional operations such as dilution and covers clinical dosage levels and pharmacokinetic (PK) studies, which can be expected to be widely applicable to antibody drug monitoring.Table 1. Fully validated antibodies by nSMOL coupled with LC-MS/MS bioanalysis. Quantitative information in human plasma for individual signature peptide sequence, MRM transition, and linear dynamic range are summarized.Antibodiesa Signature peptide sequences MRM transition Quantitation range in plasma[μg/mL]Precursor ionQ1 [m/z] Product ionQ3 [m/z]Trastuzumab IYPTNGYTR 542.8 404.7 0.06–250Bevacizumab FTFSLDTSK 523.3 797.4 0.15–300Cetuximab SQVFFK 378.2 540.3 0.58–300Rituximab ASGYTFTSYNMHWVK 598.1 817.5 0.58–300Nivolumab ASGITFSNSGMHWVR 550.8 661.5 0.15–250Brentuximab vedotin VLIYAASNLESGIPAR 837.5 343.1 0.58–300Trastuzumab emtansine IYPTNGYTR 542.8 404.7 0.06–250aDetail bioanalytical conditions for each antibody are described in: Trastuzumab (Iwamoto et al., 2015); Bevacizumab (Iwamoto, Umino, et al., 2016); Cetuximab (Iwamoto, Takanashi, et al., 2016); Rituximab (Iwamoto et al., 2016b); Nivolumab (Iwamoto, Shimada, et al., 2016); Brentuximab vedotin (Iwamoto et al., 2016a); Trastuzumab emtansine (Iwamoto et al., 2017).4. Summary and future aspectsThe analytical data obtained from MS include the structure-derived ions of measured molecules, but it can be developed into a wide variety of research applications by correctly understanding and applying the MS data. Proteomics, which is a necessary MS technology, is an optimal strategy for understanding the complicated systems biology (Bakalarski & Kirkpatrick, 2016). Although a part of the actual approach has not been generally used and applied, proteomics has become a cutting-edge scientific field, which opens new avenues for life sciences on the premise of coverage, by combining various aspects of biochemistry, analytical chemistry, physical chemistry, material science, and informatics with the structure specificity of biomolecules as an indicator. Specifically, in addition to not only structural information and molecular identification information, but also quantitative information can be obtained, which makes it possible to integrate with superior database, and it has led to great progress in understanding biology. The accumulation of quantitative proteome analysis technology is informative, and due to improvements in liquid chromatography technologies, the range of applications has dramatically expanded. The antibody drug monitoring technology nSMOL, which we recently developed, is also a technology conceived as a result of addressing the fundamental technologies of proteomics and clinical and pharmaceutical issues.Although one medicinal efficacy indicators for antibody drugs, blood concentration, is important, it is not enough. We consider that the technologies related to the estimation of lesion distribution, quantification of anti-drug antibodies and search for metabolic pathways, have the potential to open doors in research fields on the PK of antibody drugs, for which there remain many unknowns.In the future, the development of new indicators and surrogate markers is required to advance to personalized medicine and the dosing strategy of highly efficient pharmaceuticals. For the development of indicators in new fields, such as the quantification of major histocompatibility complex (MHC) peptide complex, the high efficiency identification of short chain peptides, development of exosome markers, immune cell monitoring, MS technology, and improvement of ionization reaction efficiency, will become significant areas (Caron et al., 2015, Chen and Mellman, 2013). We hope that the analytical technology of MS will expand in parallel with these advances.Conflict of interest statementAll the authors are employees of SHIMADZU Corporation, and declare no conflicts of interest associated with this manuscript.AcknowledgmentsThis review includes a part of results from research collaborations. The authors sincerely appreciate the valuable discussions with Dr. Koichi Tanaka of SHIMADZU Corporation, Dr. Masaya Ikegawa of Doshisha University, Dr. Akinobu Hamada and Dr. Hitoshi Nakagama of the National Cancer Center, and Dr. Atsushi Yonezawa of Kyoto University.ReferencesAebersold and Mann, 2016R. Aebersold, M. MannMass-spectrometric exploration of proteome structure and functionNature, 537 (2016), pp. 347-355CrossRefView Record in ScopusGoogle ScholarAnderson and Anderson, 2002N.L. Anderson, N.G. AndersonThe human plasma proteome: History, character, and diagnostic prospectsMolecular & Cellular Proteomics, 1 (2002), pp. 845-867View Record in ScopusGoogle ScholarArtimo et al., 2012P. Artimo, M. Jonnalagedda, K. Arnold, D. Baratin, G. Csardi, E. de Castro, ..., H. StockingerExPASy: SIB bioinformatics resource portalNucleic Acids Research, 40 (2012), pp. W597-603CrossRefView Record in ScopusGoogle ScholarAvallone et al., 2016A. Avallone, M.C. Piccirillo, L. Aloj, G. Nasti, P. Delrio, F. Izzo, ..., A. BudillonA randomized phase 3 study on the optimization of the combination of bevacizumab with FOLFOX/OXXEL in the treatment of patients with metastatic colorectal cancer—OBELICS (Optimization of BEvacizumab scheduLIng within Chemotherapy Scheme)BMC Cancer, 16 (2016), p. 69Google ScholarBader et al., 2017L.I. Bader, S.M. Solberg, S.H. Kaada, N. Bolstad, D.J. Warren, S. Gavasso, ..., C.A. VedelerAssays for infliximab drug levels and antibodies: A matter of scales and categoriesScandinavian Journal of Immunology, 86 (2017), pp. 165-170CrossRefView Record in ScopusGoogle ScholarBakalarski and Kirkpatrick, 2016C.E. Bakalarski, D.S. KirkpatrickA biologist's field guide to multiplexed quantitative proteomicsMolecular & Cellular Proteomics, 15 (2016), pp. 1489-1497View Record in ScopusGoogle ScholarBaselga et al., 1998J. Baselga, L. Norton, J. Albanell, Y.M. Kim, J. MendelsohnRecombinant humanized anti-HER2 antibody (Herceptin) enhances the antitumor activity of paclitaxel and doxorubicin against HER2/neu overexpressing human breast cancer xenograftsCancer Research, 58 (1998), pp. 2825-2831View Record in ScopusGoogle ScholarBeumer, 2013J.H. BeumerWithout therapeutic drug monitoring, there is no personalized cancer careClinical Pharmacology and Therapeutics, 93 (2013), pp. 228-230CrossRefView Record in ScopusGoogle ScholarCalderon-Celis et al., 2017F. Calderon-Celis, J.R. Encinar, A. Sanz-MedelStandardization approaches in absolute quantitative proteomics with mass spectrometryMass Spectrometry Reviews (2017), pp. 1-23, 10.1002/mas.21542View Record in ScopusGoogle ScholarCamon et al., 2004E. Camon, M. Magrane, D. Barrell, V. Lee, E. Dimmer, J. Maslen, ..., R. ApweilerThe Gene Ontology Annotation (GOA) database: Sharing knowledge in Uniprot with gene ontologyNucleic Acids Research, 32 (2004), pp. D262-266View Record in ScopusGoogle ScholarCaron et al., 2015E. Caron, D.J. Kowalewski, C. Chiek Koh, T. Sturm, H. Schuster, R. AebersoldAnalysis of Major Histocompatibility Complex (MHC) immunopeptidomes using mass spectrometryMolecular & Cellular Proteomics, 14 (2015), pp. 3105-3117View Record in ScopusGoogle ScholarCaron et al., 2011E. Caron, K. Vincent, M.H. Fortier, J.P. Laverdure, A. Bramoulle, M.P. Hardy, ..., C. PerreaultThe MHC I immunopeptidome conveys to the cell surface an integrative view of cellular regulationMolecular Systems Biology, 7 (2011), p. 533CrossRefGoogle ScholarChalkley et al., 2005R.J. Chalkley, P.R. Baker, L. Huang, K.C. Hansen, N.P. Allen, M. Rexach, A.L. BurlingameComprehensive analysis of a multidimensional liquid chromatography mass spectrometry dataset acquired on a quadrupole selecting, quadrupole collision cell, time-of-flight mass spectrometer: II. New developments in Protein Prospector allow for reliable and comprehensive automatic analysis of large datasetsMolecular & Cellular Proteomics, 4 (2005), pp. 1194-1204CrossRefView Record in ScopusGoogle ScholarChen and Mellman, 2013D.S. Chen, I. MellmanOncology meets immunology: The cancer-immunity cycleImmunity, 39 (2013), pp. 1-10ArticleDownload PDFCrossRefView Record in ScopusGoogle ScholarCouzin-Frankel, 2013J. Couzin-FrankelBreakthrough of the year 2013. Cancer immunotherapyScience, 342 (2013), pp. 1432-1433CrossRefView Record in ScopusGoogle ScholarCox and Mann, 2008J. Cox, M. MannMaxQuant enables high peptide identification rates, individualized p.p.b.-range mass accuracies and proteome-wide protein quantificationNature Biotechnology, 26 (2008), pp. 1367-1372CrossRefView Record in ScopusGoogle ScholarDavies and Duffield, 2017M. Davies, E.A. DuffieldSafety of checkpoint inhibitors for cancer treatment: Strategies for patient monitoring and management of immune-mediated adverse eventsImmunotargets and Therapy, 6 (2017), pp. 51-71CrossRefView Record in ScopusGoogle ScholarDeFelice et al., 2017B.C. DeFelice, S.S. Mehta, S. Samra, T. Cajka, B. Wancewicz, J.F. Fahrmann, O. FiehnMass Spectral Feature List Optimizer (MS-FLO): A tool to minimize false positive peak reports in untargeted Liquid Chromatography-Mass Spectroscopy (LC-MS) data processingAnalytical Chemistry, 89 (2017), pp. 3250-3255CrossRefView Record in ScopusGoogle ScholarDesiere et al., 2006F. Desiere, E.W. Deutsch, N.L. King, A.I. Nesvizhskii, P. Mallick, J. Eng, ..., R. AebersoldThe PeptideAtlas projectNucleic Acids Research, 34 (2006), pp. D655-658View Record in ScopusGoogle ScholarEng et al., 2015G.P. Eng, K. Bendtzen, H. Bliddal, M. Stoltenberg, M. Szkudlarek, V. Fana, ..., P.N. BoucheloucheAntibodies to infliximab and adalimumab in patients with rheumatoid arthritis in clinical remission: A cross-sectional studyArt, 2015 (2015), p. 784825View Record in ScopusGoogle ScholarEng et al., 1994J.K. Eng, A.L. McCormack, J.R. YatesAn approach to correlate tandem mass spectral data of peptides with amino acid sequences in a protein databaseJournal of the American Society for Mass Spectrometry, 5 (1994), pp. 976-989ArticleDownload PDFCrossRefView Record in ScopusGoogle ScholarFrancavilla et al., 2017C. Francavilla, M. Lupia, K. Tsafou, A. Villa, K. Kowalczyk, R. Rakownikow Jersie-Christensen, ..., J.V. OlsenPhosphoproteomics of primary cells reveals druggable kinase signatures in ovarian cancerCell Reports, 18 (2017), pp. 3242-3256ArticleDownload PDFView Record in ScopusGoogle ScholarFukuyama et al., 2008Y. Fukuyama, S. Nakaya, Y. Yamazaki, K. TanakaIonic liquid matrixes optimized for MALDI-MS of sulfated/sialylated/neutral oligosaccharides and glycopeptidesAnalytical Chemistry, 80 (2008), pp. 2171-2179CrossRefView Record in ScopusGoogle ScholarGao et al., 2012B. Gao, S. Yeap, A. Clements, B. Balakrishnar, M. Wong, H. GurneyEvidence for therapeutic drug monitoring of targeted anticancer therapiesJournal of Clinical Oncology, 30 (2012), pp. 4017-4025View Record in ScopusGoogle ScholarGhaste et al., 2016M. Ghaste, R. Mistrik, V. ShulaevApplications of Fourier Transform Ion Cyclotron Resonance (FT-ICR) and orbitrap based high resolution mass spectrometry in metabolomics and lipidomicsInternational Journal of Molecular Sciences, 17 (2016)Google ScholarGiansanti et al., 2016P. Giansanti, L. Tsiatsiani, T.Y. Low, A.J. HeckSix alternative proteases for mass spectrometry-based proteomics beyond trypsinNature Protocols, 11 (2016), pp. 993-1006CrossRefView Record in ScopusGoogle ScholarGoshima et al., 2008N. Goshima, Y. Kawamura, A. Fukumoto, A. Miura, R. Honma, R. Satoh, ..., N. NomuraHuman protein factory for converting the transcriptome into an in vitro-expressed proteomeNature Methods, 5 (2008), pp. 1011-1017CrossRefView Record in ScopusGoogle ScholarGuthals and Bandeira, 2012A. Guthals, N. BandeiraPeptide identification by tandem mass spectrometry with alternate fragmentation modesMolecular & Cellular Proteomics, 11 (2012), pp. 550-557View Record in ScopusGoogle ScholarHamada et al., 2003A. Hamada, H. Miyano, H. Watanabe, H. SaitoInteraction of imatinib mesilate with human P-glycoproteinThe Journal of Pharmacology and Experimental Therapeutics, 307 (2003), pp. 824-828View Record in ScopusGoogle ScholarHardman and Makarov, 2003M. Hardman, A.A. MakarovInterfacing the orbitrap mass analyzer to an electrospray ion sourceAnalytical Chemistry, 75 (2003), pp. 1699-1705View Record in ScopusGoogle ScholarHommerson et al., 2011P. Hommerson, A.M. Khan, G.J. de Jong, G.W. SomsenIonization techniques in capillary electrophoresis-mass spectrometry: Principles, design, and applicationMass Spectrometry Reviews, 30 (2011), pp. 1096-1120CrossRefView Record in ScopusGoogle ScholarHowden et al., 2013A.J. Howden, V. Geoghegan, K. Katsch, G. Efstathiou, B. Bhushan, O. Boutureira, ..., O. AcutoQuaNCAT: Quantitating proteome dynamics in primary cellsNature Methods, 10 (2013), pp. 343-346CrossRefView Record in ScopusGoogle ScholarHowells et al., 2005L.C. Howells, A.O. Whelan, M.J. Woodward, N.G. ColdhamSimple and effective technical procedures for the packing and application of nanospray PicoFrit HPLC columns for proteomic analysesProteomics, 5 (2005), pp. 3864-3867CrossRefView Record in ScopusGoogle ScholarHuang da et al., 2009W. Huang da, B.T. Sherman, R.A. LempickiSystematic and integrative analysis of large gene lists using DAVID bioinformatics resourcesNature Protocols, 4 (2009), pp. 44-57View Record in ScopusGoogle ScholarIshida et al., 1992Y. Ishida, Y. Agata, K. Shibahara, T. HonjoInduced expression of PD-1, a novel member of the immunoglobulin gene superfamily, upon programmed cell deathEMBO Journal, 11 (1992), pp. 3887-3895View Record in ScopusGoogle ScholarIsmaiel et al., 2017O.A. Ismaiel, W.R. Mylott Jr., R.G. JenkinsDo we have a mature LC-MS/MS methodology for therapeutic monoclonal antibody bioanalysis?Bioanalysis, 9 (2017), pp. 1289-1292CrossRefView Record in ScopusGoogle ScholarIto et al., 2015A. Ito, S. Kondo, K. Tada, S. KitanoClinical development of immune checkpoint inhibitorsBioMed Research International, 2015 (2015), p. 605478Google ScholarIwamoto et al., 2017N. Iwamoto, A. Hamada, T. ShimadaAntibody drug quantitation in coexistence with anti-drug antibodies on nSMOL bioanalysisAnalytical Biochemistry, 540-541 (2017), pp. 30-37Google ScholarIwamoto et al., 2016N. Iwamoto, T. Shimada, H. Terakado, A. HamadaValidated LC-MS/MS analysis of immune checkpoint inhibitor Nivolumab in human plasma using a Fab peptide-selective quantitation method: Nano-surface and molecular-orientation limited (nSMOL) proteolysisJournal of Chromatography. B, Analytical Technologies in the Biomedical and Life Sciences, 1023-1024 (2016), pp. 9-16ArticleDownload PDFView Record in ScopusGoogle ScholarIwamoto et al., 2014N. Iwamoto, T. Shimada, Y. Umino, C. Aoki, Y. Aoki, T.A. Sato, ..., H. NakagamaSelective detection of complementarity-determining regions of monoclonal antibody by limiting protease access to the substrate: Nano-surface and molecular-orientation limited proteolysisAnalyst, 139 (2014), pp. 576-580CrossRefGoogle ScholarIwamoto et al., 2017N. Iwamoto, A. Shimomura, K. Tamura, A. Hamada, T. ShimadaLC-MS bioanalysis of Trastuzumab and released emtansine using nano-surface and molecular-orientation limited (nSMOL) proteolysis and liquid-liquid partition in plasma of Trastuzumab emtansine-treated breast cancer patientsJournal of Pharmaceutical and Biomedical Analysis, 145 (2017), pp. 33-39ArticleDownload PDFView Record in ScopusGoogle ScholarIwamoto et al., 2016dN. Iwamoto, M. Takanashi, A. Hamada, T. ShimadaMultiplex LCMS bioanalysis of Brentuximab Vedotin, Rituximab and Cetuximab towards therapeutic drug monitoring application by combined calibration curve using Fab-selective limited proteolysis nSMOLClinical Pharmacology & Biopharmaceutics, 5 (2016)Google ScholarIwamoto et al., 2016eN. Iwamoto, M. Takanashi, A. Hamada, T. ShimadaValidated LC/MS bioanalysis of Rituximab CDR peptides using nano-surface and Molecular-Orientation Limited (nSMOL) proteolysisBiological & Pharmaceutical Bulletin, 39 (2016), pp. 1187-1194CrossRefView Record in ScopusGoogle ScholarIwamoto et al., 2016N. Iwamoto, M. Takanashi, Y. Umino, C. Aoki, A. Hamada, T. ShimadaApplication of nano-surface and molecular-orientation limited proteolysis to LC-MS bioanalysis of cetuximabBioanalysis, 8 (2016), pp. 1009-1020CrossRefView Record in ScopusGoogle ScholarIwamoto et al., 2016N. Iwamoto, Y. Umino, C. Aoki, N. Yamane, A. Hamada, T. ShimadaFully validated LCMS bioanalysis of Bevacizumab in human plasma using nano-surface and molecular-orientation limited (nSMOL) proteolysisDrug Metabolism and Pharmacokinetics, 31 (2016), pp. 46-50ArticleDownload PDFView Record in ScopusGoogle ScholarIwamoto et al., 2015N. Iwamoto, N. Yamane, Y. Umino, A. Hamada, T. ShimadaDevelopment of the validated LCMS bioanalysis of Trastuzumab in human plasma using selective detection method for complementarity-determining regions of monoclonal antibody: Nano-surface and molecular-orientation limited (nSMOL) proteolysisAnalytical Methods, 7 (2015), pp. 9177-9183CrossRefView Record in ScopusGoogle ScholarIwasaki et al., 2010M. Iwasaki, S. Miwa, T. Ikegami, M. Tomita, N. Tanaka, Y. IshihamaOne-dimensional capillary liquid chromatographic separation coupled with tandem mass spectrometry unveils the Escherichia coli proteome on a microarray scaleAnalytical Chemistry, 82 (2010), pp. 2616-2620CrossRefView Record in ScopusGoogle ScholarJingushi et al., 2017K. Jingushi, M. Uemura, N. Ohnishi, W. Nakata, K. Fujita, T. Naito, ..., K. UedaExtracellular vesicles isolated from human renal cell carcinoma tissues disrupt vascular endothelial cell morphology via azurocidinInternational Journal of Cancer, 142 (3) (2017), pp. 607-617Google ScholarKang et al., 2014Y.K. Kang, S.Y. Rha, P. Tassone, J. Barriuso, R. Yu, T. Szado, ..., Y.J. BangA phase IIa dose-finding and safety study of first-line pertuzumab in combination with trastuzumab, capecitabine and cisplatin in patients with HER2-positive advanced gastric cancerBritish Journal of Cancer, 111 (2014), pp. 660-666CrossRefView Record in ScopusGoogle ScholarKirley et al., 2016T.L. Kirley, K.D. Greis, A.B. NormanStructural characterization of expressed monoclonal antibodies by single sample mass spectral analysis after IdeS proteolysisBiochemical and Biophysical Research Communications, 477 (2016), pp. 363-368ArticleDownload PDFView Record in ScopusGoogle ScholarKreimer et al., 2017S. Kreimer, Y. Gao, S. Ray, M. Jin, Z. Tan, N.A. Mussa, ..., B.L. KargerHost cell protein profiling by targeted and untargeted analysis of data independent acquisition mass spectrometry data with parallel reaction monitoring verificationAnalytical Chemistry, 89 (2017), pp. 5294-5302CrossRefView Record in ScopusGoogle ScholarKurachi et al., 2009S. Kurachi, J.S. Huo, A. Ameri, K. Zhang, A.C. Yoshizawa, K. KurachiAn age-related homeostasis mechanism is essential for spontaneous amelioration of hemophilia B LeydenProceedings of the National Academy of Sciences of the United States of America, 106 (2009), pp. 7921-7926CrossRefView Record in ScopusGoogle ScholarLam et al., 2007T.C. Lam, K.K. Li, S.C. Lo, J.A. Guggenheim, C.H. ToApplication of fluorescence difference gel electrophoresis technology in searching for protein biomarkers in chick myopiaJournal of Proteome Research, 6 (2007), pp. 4135-4149CrossRefView Record in ScopusGoogle ScholarLapek Jr. et al., 2017J.D. Lapek Jr., P. Greninger, R. Morris, A. Amzallag, I. Pruteanu-Malinici, C.H. Benes, W. HaasDetection of dysregulated protein-association networks by high-throughput proteomics predicts cancer vulnerabilitiesNature Biotechnology, 35 (2017), pp. 983-989CrossRefView Record in ScopusGoogle ScholarLiu et al., 2012Y. Liu, Z. Miao, R. Lakshmanan, R.R. Ogorzalek Loo, J.A. Loo, H. ChenSignal and charge enhancement for protein analysis by liquid chromatography-mass spectrometry with desorption electrospray ionizationInternational Journal of Mass Spectrometry, 325-327 (2012), pp. 161-166ArticleDownload PDFView Record in ScopusGoogle ScholarMacLean et al., 2010B. MacLean, D.M. Tomazela, N. Shulman, M. Chambers, G.L. Finney, B. Frewen, ..., M.J. MacCossSkyline: An open source document editor for creating and analyzing targeted proteomics experimentsBioinformatics, 26 (2010), pp. 966-968CrossRefView Record in ScopusGoogle ScholarMaloney et al., 1997D.G. Maloney, A.J. Grillo-Lopez, C.A. White, D. Bodkin, R.J. Schilder, J.A. Neidhart, ..., R. LevyIDEC-C2B8 (Rituximab) anti-CD20 monoclonal antibody therapy in patients with relapsed low-grade non-Hodgkin's lymphomaBlood, 90 (1997), pp. 2188-2195View Record in ScopusGoogle ScholarMarinari et al., 2014B. Marinari, E. Botti, M. Bavetta, G. Spallone, A. Zangrilli, M. Talamonti, ..., A. CostanzoDetection of adalimumab and anti-adalimumab levels by ELISA: Clinical considerationsDrug Development Research, 75 (Suppl. 1) (2014), pp. S11-14CrossRefView Record in ScopusGoogle ScholarMartin et al., 2007F.P. Martin, M.E. Dumas, Y. Wang, C. Legido-Quigley, I.K. Yap, H. Tang, ..., J.K. NicholsonA top-down systems biology view of microbiome-mammalian metabolic interactions in a mouse modelMolecular Systems Biology, 3 (2007), p. 112CrossRefGoogle ScholarMatsumoto et al., 2017M. Matsumoto, F. Matsuzaki, K. Oshikawa, N. Goshima, M. Mori, Y. Kawamura, ..., K.I. NakayamaA large-scale targeted proteomics assay resource based on an in vitro human proteomeNature Methods, 14 (2017), pp. 251-258CrossRefView Record in ScopusGoogle ScholarMessenheimer et al., 2017D.J. Messenheimer, S.M. Jensen, M.E. Afentoulis, K.W. Wegmann, Z. Feng, D.J. Friedman, ..., B.A. FoxTiming of PD-1 blockade is critical to effective combination immunotherapy with anti-OX40Clinical Cancer Research, 23 (2017), pp. 6165-6177View Record in ScopusGoogle ScholarMichalski et al., 2011A. Michalski, J. Cox, M. MannMore than 100,000 detectable peptide species elute in single shotgun proteomics runs but the majority is inaccessible to data-dependent LC-MS/MSJournal of Proteome Research, 10 (2011), pp. 1785-1793CrossRefView Record in ScopusGoogle ScholarMora et al., 2000J.F. Mora, G.J. Van Berkel, C.G. Enke, R.B. Cole, M. Martinez-Sanchez, J.B. FennElectrochemical processes in electrospray ionization mass spectrometryJournal of Mass Spectrometry, 35 (2000), pp. 939-952CrossRefGoogle ScholarMorris et al., 1982H.R. Morris, M. Panico, A. Karplus, P.E. Lloyd, B. RinikerElucidation by FAB-MS of the structure of a new cardioactive peptide from AplysiaNature, 300 (1982), pp. 643-645CrossRefView Record in ScopusGoogle ScholarMullard, 2017aA. Mullard2016 FDA drug approvalsNature Reviews. Drug Discovery, 16 (2017), pp. 73-76CrossRefView Record in ScopusGoogle ScholarMullard, 2017bA. MullardBispecific antibody pipeline moves beyond oncologyNature Reviews. Drug Discovery, 16 (11) (2017), p. 810CrossRefGoogle ScholarMulleman et al., 2009D. Mulleman, J.C. Meric, G. Paintaud, E. Ducourau, C. Magdelaine-Beuzelin, J.P. Valat, ..., Centre National de la Recherche Scientifique, U. GInfliximab concentration monitoring improves the control of disease activity in rheumatoid arthritisArthritis Research & Therapy, 11 (2009), p. R178CrossRefGoogle ScholarNirmalan et al., 2011N.J. Nirmalan, C. Hughes, J. Peng, T. McKenna, J. Langridge, D.A. Cairns, ..., R.E. BanksInitial development and validation of a novel extraction method for quantitative mining of the formalin-fixed, paraffin-embedded tissue proteome for biomarker investigationsJournal of Proteome Research, 10 (2011), pp. 896-906CrossRefGoogle ScholarNovotny and Bruccoleri, 1987J. Novotny, R.E. BruccoleriCorrelation among sites of limited proteolysis, enzyme accessibility and segmental mobilityFEBS Letters, 211 (1987), pp. 185-189ArticleDownload PDFCrossRefView Record in ScopusGoogle ScholarOka et al., 1991H. Oka, Y. Ikai, N. Kawamura, J. Hayakawa, K. Harada, H. Murata, ..., Y. ItoDirect interfacing of high-speed countercurrent chromatography to frit electron ionization, chemical ionization, and fast atom bombardment mass spectrometryAnalytical Chemistry, 63 (1991), pp. 2861-2865CrossRefView Record in ScopusGoogle ScholarOng et al., 2002S.E. Ong, B. Blagoev, I. Kratchmarova, D.B. Kristensen, H. Steen, A. Pandey, M. MannStable isotope labeling by amino acids in cell culture, SILAC, as a simple and accurate approach to expression proteomicsMolecular & Cellular Proteomics, 1 (2002), pp. 376-386CrossRefView Record in ScopusGoogle ScholarPappin et al., 1993D.J. Pappin, P. Hojrup, A.J. BleasbyRapid identification of proteins by peptide-mass fingerprintingCurrent Biology, 3 (1993), pp. 327-332ArticleDownload PDFView Record in ScopusGoogle ScholarPoste et al., 2012G. Poste, D.P. Carbone, D.R. Parkinson, J. Verweij, S.M. Hewitt, J.M. JessupLeveling the playing field: Bringing development of biomarkers and molecular diagnostics up to the standards for drug developmentClinical Cancer Research, 18 (2012), pp. 1515-1523CrossRefView Record in ScopusGoogle ScholarPouw et al., 2015M.F. Pouw, C.L. Krieckaert, M.T. Nurmohamed, D. van der Kleij, L. Aarden, T. Rispens, G. WolbinkKey findings towards optimising adalimumab treatment: The concentration-effect curveAnnals of the Rheumatic Diseases, 74 (2015), pp. 513-518CrossRefView Record in ScopusGoogle ScholarPrzybylski et al., 1982M. Przybylski, J. Preiss, R. Dennebaum, J. FischerIdentification and quantitation of methotrexate and methotrexate metabolites in clinical high-dose therapy by high pressure liquid chromatography and field desorption mass spectrometryBiomedical Mass Spectrometry, 9 (1982), pp. 22-32CrossRefView Record in ScopusGoogle ScholarRamani et al., 2008A.K. Ramani, Z. Li, G.T. Hart, M.W. Carlson, D.R. Boutz, E.M. MarcotteA map of human protein interactions derived from co-expression of human mRNAs and their orthologsMolecular Systems Biology, 4 (2008), p. 180CrossRefGoogle ScholarRazavi et al., 2012M. Razavi, L.E. Frick, W.A. LaMarr, M.E. Pope, C.A. Miller, N.L. Anderson, T.W. PearsonHigh-throughput SISCAPA quantitation of peptides from human plasma digests by ultrafast, liquid chromatography-free mass spectrometryJournal of Proteome Research, 11 (2012), pp. 5642-5649CrossRefView Record in ScopusGoogle ScholarRifai et al., 2006N. Rifai, M.A. Gillette, S.A. CarrProtein biomarker discovery and validation: The long and uncertain path to clinical utilityNature Biotechnology, 24 (2006), pp. 971-983CrossRefView Record in ScopusGoogle ScholarSelevsek et al., 2015N. Selevsek, C.Y. Chang, L.C. Gillet, P. Navarro, O.M. Bernhardt, L. Reiter, ..., R. AebersoldReproducible and consistent quantification of the Saccharomyces cerevisiae proteome by SWATH-mass spectrometryMolecular & Cellular Proteomics, 14 (2015), pp. 739-749View Record in ScopusGoogle ScholarShimada et al., 2010T. Shimada, T. Nakanishi, A. Toyama, S. Yamauchi, A. Kanzaki, H. Fujiwake, ..., M. IkegawaPotential implications for monitoring serum bile acid profiles in circulation with serum proteome for carbon tetrachloride-induced liver injury/regeneration model in miceJournal of Proteome Research, 9 (2010), pp. 4490-4500CrossRefView Record in ScopusGoogle ScholarStanding, 2003K.G. StandingPeptide and protein de novo sequencing by mass spectrometryCurrent Opinion in Structural Biology, 13 (2003), pp. 595-601ArticleDownload PDFView Record in ScopusGoogle ScholarSteenholdt et al., 2014C. Steenholdt, K. Bendtzen, J. Brynskov, O.O. Thomsen, M.A. AinsworthClinical implications of measuring drug and anti-drug antibodies by different assays when optimizing infliximab treatment failure in Crohn's disease: Post hoc analysis of a randomized controlled trialThe American Journal of Gastroenterology, 109 (2014), pp. 1055-1064CrossRefView Record in ScopusGoogle ScholarStephanowitz et al., 2012H. Stephanowitz, S. Lange, D. Lang, C. Freund, E. KrauseImproved two-dimensional reversed phase-reversed phase LC-MS/MS approach for identification of peptide-protein interactionsJournal of Proteome Research, 11 (2012), pp. 1175-1183CrossRefView Record in ScopusGoogle ScholarSun et al., 2014L. Sun, A.S. Hebert, X. Yan, Y. Zhao, M.S. Westphall, M.J. Rush, ..., N.J. DovichiOver 10,000 peptide identifications from the HeLa proteome by using single-shot capillary zone electrophoresis combined with tandem mass spectrometryAngewandte Chemie (International Ed. in English), 53 (2014), pp. 13931-13933CrossRefView Record in ScopusGoogle ScholarSwaney et al., 2010D.L. Swaney, C.D. Wenger, J.J. CoonValue of using multiple proteases for large-scale mass spectrometry-based proteomicsJournal of Proteome Research, 9 (2010), pp. 1323-1329CrossRefView Record in ScopusGoogle ScholarTabernero et al., 2017J. Tabernero, A. Ohtsu, K. Muro, E. Van Cutsem, S.C. Oh, G. Bodoky, ..., C.S. FuchsExposure-response analyses of Ramucirumab from two randomized, phase III trials of second-line treatment for advanced gastric or gastroesophageal junction cancerMolecular Cancer Therapeutics, 16 (2017), pp. 2215-2222View Record in ScopusGoogle ScholarTargan et al., 1997S.R. Targan, S.B. Hanauer, S.J. van Deventer, L. Mayer, D.H. Present, T. Braakman, ..., P.J. RutgeertsA short-term study of chimeric monoclonal antibody cA2 to tumor necrosis factor alpha for Crohn's disease. Crohn's Disease cA2 Study GroupThe New England Journal of Medicine, 337 (1997), pp. 1029-1035View Record in ScopusGoogle ScholarTrenchevska et al., 2016O. Trenchevska, R.W. Nelson, D. NedelkovMass spectrometric immunoassays for discovery, screening and quantification of clinically relevant proteoformsBioanalysis, 8 (2016), pp. 1623-1633CrossRefView Record in ScopusGoogle ScholarUeda et al., 2011K. Ueda, N. Saichi, S. Takami, D. Kang, A. Toyama, Y. Daigo, ..., H. NakagawaA comprehensive peptidome profiling technology for the identification of early detection biomarkers for lung adenocarcinomaPLoS One, 6 (2011), Article e18567CrossRefGoogle ScholarUhlen et al., 2015M. Uhlen, L. Fagerberg, B.M. Hallstrom, C. Lindskog, P. Oksvold, A. Mardinoglu, ..., F. PontenProteomics. Tissue-based map of the human proteomeScience, 347 (2015), p. 1260419CrossRefGoogle ScholarUhlen et al., 2010M. Uhlen, P. Oksvold, L. Fagerberg, E. Lundberg, K. Jonasson, M. Forsberg, ..., F. PontenTowards a knowledge-based human protein atlasNature Biotechnology, 28 (2010), pp. 1248-1250CrossRefView Record in ScopusGoogle ScholarValkenborg et al., 2008D. Valkenborg, I. Jansen, T. BurzykowskiA model-based method for the prediction of the isotopic distribution of peptidesJournal of the American Society for Mass Spectrometry, 19 (2008), pp. 703-712ArticleDownload PDFCrossRefView Record in ScopusGoogle ScholarVasan, 2006R.S. VasanBiomarkers of cardiovascular disease: Molecular basis and practical considerationsCirculation, 113 (2006), pp. 2335-2362View Record in ScopusGoogle ScholarWong, 2001S.H. WongTherapeutic drug monitoring for immunosuppressantsClinica Chimica Acta, 313 (2001), pp. 241-253ArticleDownload PDFView Record in ScopusGoogle ScholarWu and Kabat, 1970T.T. Wu, E.A. KabatAn analysis of the sequences of the variable regions of Bence Jones proteins and myeloma light chains and their implications for antibody complementarityThe Journal of Experimental Medicine, 132 (1970), pp. 211-250CrossRefView Record in ScopusGoogle ScholarZeeberg et al., 2003B.R. Zeeberg, W. Feng, G. Wang, M.D. Wang, A.T. Fojo, M. Sunshine, ..., J.N. WeinsteinGoMine
- Home >
- Catalog >
- Education >
- Chemistry Chart >
- Electron Configuration Chart >
- Electron Configuration And The Periodic Table >
- Electron Configuration In Ionic Bonding Section Review