How to Edit and fill out Determine Whether The Ratios Are Equivalent 3 10 And 6 20 Online
Read the following instructions to use CocoDoc to start editing and writing your Determine Whether The Ratios Are Equivalent 3 10 And 6 20:
- In the beginning, find the “Get Form” button and press it.
- Wait until Determine Whether The Ratios Are Equivalent 3 10 And 6 20 is appeared.
- Customize your document by using the toolbar on the top.
- Download your completed form and share it as you needed.
An Easy-to-Use Editing Tool for Modifying Determine Whether The Ratios Are Equivalent 3 10 And 6 20 on Your Way
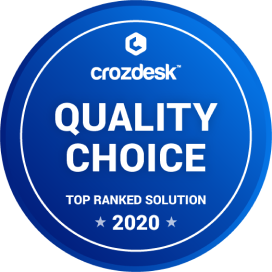
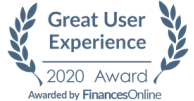
Open Your Determine Whether The Ratios Are Equivalent 3 10 And 6 20 Without Hassle
Get FormHow to Edit Your PDF Determine Whether The Ratios Are Equivalent 3 10 And 6 20 Online
Editing your form online is quite effortless. You don't need to download any software on your computer or phone to use this feature. CocoDoc offers an easy tool to edit your document directly through any web browser you use. The entire interface is well-organized.
Follow the step-by-step guide below to eidt your PDF files online:
- Search CocoDoc official website on your device where you have your file.
- Seek the ‘Edit PDF Online’ icon and press it.
- Then you will browse this page. Just drag and drop the template, or append the file through the ‘Choose File’ option.
- Once the document is uploaded, you can edit it using the toolbar as you needed.
- When the modification is finished, tap the ‘Download’ option to save the file.
How to Edit Determine Whether The Ratios Are Equivalent 3 10 And 6 20 on Windows
Windows is the most widely-used operating system. However, Windows does not contain any default application that can directly edit template. In this case, you can download CocoDoc's desktop software for Windows, which can help you to work on documents easily.
All you have to do is follow the instructions below:
- Download CocoDoc software from your Windows Store.
- Open the software and then choose your PDF document.
- You can also choose the PDF file from Dropbox.
- After that, edit the document as you needed by using the varied tools on the top.
- Once done, you can now save the completed PDF to your computer. You can also check more details about the best way to edit PDF.
How to Edit Determine Whether The Ratios Are Equivalent 3 10 And 6 20 on Mac
macOS comes with a default feature - Preview, to open PDF files. Although Mac users can view PDF files and even mark text on it, it does not support editing. Through CocoDoc, you can edit your document on Mac directly.
Follow the effortless guidelines below to start editing:
- To start with, install CocoDoc desktop app on your Mac computer.
- Then, choose your PDF file through the app.
- You can select the template from any cloud storage, such as Dropbox, Google Drive, or OneDrive.
- Edit, fill and sign your file by utilizing this CocoDoc tool.
- Lastly, download the template to save it on your device.
How to Edit PDF Determine Whether The Ratios Are Equivalent 3 10 And 6 20 on G Suite
G Suite is a widely-used Google's suite of intelligent apps, which is designed to make your workforce more productive and increase collaboration with each other. Integrating CocoDoc's PDF editing tool with G Suite can help to accomplish work easily.
Here are the instructions to do it:
- Open Google WorkPlace Marketplace on your laptop.
- Search for CocoDoc PDF Editor and get the add-on.
- Select the template that you want to edit and find CocoDoc PDF Editor by selecting "Open with" in Drive.
- Edit and sign your file using the toolbar.
- Save the completed PDF file on your device.
PDF Editor FAQ
Can the two terms of a ratio be in two different units?
Ratios and ProportionsRatios are used to compare quantities. Ratios help us to compare quantities and determine the relation between them. A ratio is a comparison of two similar quantities obtained by dividing one quantity by the other. Since a ratio is only a comparison or relation between quantities, it is an abstract number. For instance, the ratio of 6 miles to 3 miles is only 2, not 2 miles. Ratios are written with the” : “symbol.If two quantities cannot be expressed in terms of the same unit, there cannot be a ratio between them. Hence to compare two quantities, the units must be the same.Consider an example to find the ratio of 3 km to 300 m.First convert both the distances to the same unit.So, 3 km = 3 × 1000 m = 3000 m.Thus, the required ratio, 3 km : 300 m is 3000 : 300 = 10 : 1Equivalent RatiosDifferent ratios can also be compared with each other to know whether they are equivalent or not. To do this, we need to write the ratios in the form of fractions and then compare them by converting them to like fractions. If these like fractions are equal, we say the given ratios are equivalent. We can find equivalent ratios by multiplying or dividing the numerator and denominator by the same number. Consider an example to check whether the ratios 1 : 2 and 2 : 3 equivalent.To check this, we need to know whetherWe have,We find thatwhich means thatTherefore, the ratio 1 :2 is not equivalent to the ratio 2 : 3.ProportionThe ratio of two quantities in the same unit is a fraction that shows how many times one quantity is greater or smaller than the other. Four quantities are said to be in proportion, if the ratio of first and second quantities is equal to the ratio of third and fourth quantities. If two ratios are equal, then we say that they are in proportion and use the symbol ‘:: ’ or ‘=’ to equate the two ratios.Solving Ratio and ProportionRatio and proportion problems can be solved by using two methods, the unitary method and equating the ratios to make proportions, and then solving the equation.For example,To check whether 8, 22, 12, and 33 are in proportion or not, we have to find the ratio of 8 to 22 and the ratio of 12 to 33.Therefore, 8, 22, 12, and 33 are in proportion as 8 : 22 and 12 : 33 are equal. When four terms are in proportion, the first and fourth terms are known as extreme terms and the second and third terms are known as middle terms. In the above example, 8, 22, 12, and 33 were in proportion. Therefore, 8 and 33 are known as extreme terms while 22 and 12 are known as middle terms.The method in which we first find the value of one unit and then the value of the required number of units is known as unitary method.Consider an example to find the cost of 9 bananas if the cost of a dozen bananas is Rs 20.1 dozen = 12 unitsCost of 12 bananas = Rs 20∴ Cost of 1 bananas = Rs∴ Cost of 9 bananas = RsThis method is known as unitary method.SummaryWe have learnt, Ratios are used to compare quantities.Since a ratio is only a comparison or relation between quantities, it is an abstract number.Ratios can be written as fractions. They also have all the properties of fractions.The ratio of 6 to 3 should be stated as 2 to 1, but common usage has shortened the expression of ratios to be called simply 2.If two quantities cannot be expressed in terms of the same unit, there cannot be a ratio between them.If any three terms in a proportion are given, the fourth may be found. The product of the means is equal to the product of the extremes.It is important to remember that to use the proportion; the ratios must be equal to each other and must remain constant.link :Ratios and Proportions
Why does the discriminant determine the number of solutions?
First of all, I am sorry if I don’t write in great English, or if I mess up some math terminology, I am not used to speaking about math in English, I don’t know all the math terminology, but I hope I will be able to help. If you are unfamiliar with linear algebra, feel free to skip the first part of my answer and go straight to the example that I gave.I suspect you mean solutions to a system of equations, or do you mean the number of vectors which give you a certain resulting vector after a matrix transformation. It doesn’t matter actually, since these two questions are equivalent. If the determinant is zero then infinitely many vectors (or infinitely many values for x and y, if you are dealing with a system of 2 equations) are solutions or no vector (no values for x and y) is the solution. Why is this the case ? Because the vector space collapses when the determinant is zero, the rank of the vector space goes down. Why ? because determinant is the measure of how much the vector space got stretched, determinant is the factor by which the area, volume or hypervolume formed by unit vectors got multiplied. If determinant is 0 that means that area/volume/hypervolume formed by unit vectors got multiplied by 0 which means that vector space now has smaller number of dimensions. If vector space was 2 dimensional, now it is 1 dimensional (a line) or 0 dimensional (a dot) which means that if the vector that you need to get is not on that line or it isn’t a zero vector in the latter case, then no vector is a solution to the problem. Let me give you an example.Let’s say you have a system of equations whose determinant is zero2x + 6y = 104x + 12y = 20Another way to set up the same problem as this system of two equations is to say the following:when the matrix2 64 12is applied to the vectorxyyou will get the resulting vector1020This is another way to formulate the same mathematical problem.Now let’s get back to the equations2x + 6y = 104x + 12y = 20Just copy the first equation and manipulate the other2x + 6y = 102(2x + 6y) = 202x + 6y = 102x + 6y = 10You got one equation with two variables, which means there are infinitely many solutions, in fact, all of them lie on the same line2x+6y=106y=10–2x6y=2(5–x)y= - x/3 + 5/3.System of equations whose determinant is 0 can either be reduced to a system which has more variables then equations, which means that such system can’t have a single solution (it has infinitely many solutions), either it doesn't have any solution at all. Notice that 4x + 12y can be rewritten as 2(2x+6y). That’s the same equation multiplied by a factor of 2. Now, the only reason why there is any solution at all is because the ratio at the right hand side of the equations, namely 10/20 is the same as the ratio of the left hand side of the equations, namely (2x+6y) / (2(2x+6y)) = 1/2. Left hand side of the equations gives you the same ratio as the right hand side of the equations. If the left hand side and the right hand side didn’t give you the same ratio, there would be no solution to this system of equations, there would be no values for x and y that satisfy the system of equations.Here’s another way to check whether the ratio of the left hand side of the equations is the same as the ratio of the right hand side of the equations.Let p = 2x + 6y,then 2p = 4x + 12yIf you replace p into the original system of equations you will getp = 102p = 20The ratio of left hand side p/(2p) is equal to the ratio of the right hand side 10/20.If that wasn’t the case, there would be no solutions to the system of equations.For instance, if you had a system of equations2x + 6y = 104x + 12y = 50there would be no solutions for this system of equations because the ratio of the left hand side is 1/2 and the ratio of the right hand side is 1/5.If you try to solve it, you will get2x + 6y = 102(2x + 6y) = 502x + 6y = 102*10 = 50 which is a contradiction, which means that there is no solution to this system of equations.This has a very nice and intuitive explanation in linear algebra (again, feel free to stop reading the rest of my comment if you are not familiar with linear algebra).If you apply a matrix transformation which reduces the vector space to a line, then the resulting vector must be on that line if you want to have any solution at all, because all the vectors will end up on that line when this matrix transformation is applied to them, there is no way for any vector to end up anywhere outside that line.What does this have to do with the ratio of left hand side of the equations being equal to the ratio of the right hand side of the equations ?Shorter answer: The ratio of the left hand side of the equations determines the line to which vector space gets reduced. The ratio of the right hand side of the equations determines the line on which the resulting vector is (the resulting vector is the vector that you get after matrix transformation is applied to the x y vector). If the ratio of the left hand side of the equations is different to the ratio of the right hand side of the equations, the resulting vector is not on the line to which the vector space gets reduced (the line to which vector space gets reduced and the line to which resulting vector belongs are 2 different lines). If the resulting vector is not on the line to which vector space gets reduced, there is no solution to the problem, there is no vector x y that becomes the resulting vector after matrix transformation is applied to it.Longer answer:Well, a line in mathematics is described by a linear function y=kx+n (if you wonder why that's the case, it is because a line never changes its direction, it goes straight, which means that a line has a constant angle with respect to x axis, which means that its first derivative with respect to x is a constant). Since the line to which vector space gets reduced passes through unit vectors and since the unit vectors don’t change their origin point (0,0) after any matrix transformation, the line to which the vector space gets reduced is a line which is described by a function y=kx + n and n = 0, because the line crosses the origin point (0,0).The equation for the line to which vector space gets reduced is therefore just y = kx. The line is therefore totally determined by k, which is equal to y/x.So, you know that the line to which vector space gets reduced has the same ratio k = y/x as the unit vectors do after matrix transformation (the reason why vector space got reduced to a line in the first place is because both unit vectors ended up on the same line after matrix transformation was applied, which means that all the vectors that you can construct by adding unit vectors lie on the same line. No matter how you add unit vectors, you will always stay on the same line. However you combine the unit vectors, you cannot escape the line on which they are on. All the vectors that you can construct by adding unit vectors are on the same line).The ratio of the x coeficients in the left hand side of the equations ( 4/2 in our example) is the k = y/x ratio of the first unit vector and the ratio of y coeficients in the left hand side of the equation (12/6 in our example) is the k = y/x ratio of the other unit vector. In our case, as I have already said, the vector space gets reduced to a line and both unit vectors lie on it, which means that both unit vectors as well as the line on which they lie on have the same k = y/x ratio. If both unit vectors belong to the same line that means the ratio of x coeficients is the same as the ratio of y coefficients (which is 4/2 = 12/6 = 2 in our example). Since the vector space gets reduced to a line whose k = y/x ratio is equal to 2, all vectors will have the same y/x =2 ratio after matrix transformation is applied to them. They will all lie on the line y=2x.Now, the right hand side of our equations is the resulting vector. If its ratio k=y/x is 2, that vector lies on the same line that vector space gets reduced to and therefore there will be solutions. If, however, its ratio k=y/x is not 2, then the resulting vector does not belong to the line to which the vector space gets reduced after matrix transformation and therefore, there is no solution, because there is no vector which will end up outside of the line to which the vector space gets reduced after matrix transformation is applied to it.Let’s go through all of this with our example, which will make it easier to understand.Our matrix2 64 12reduces the vector space to a line y = 2x.The resulting vector (10,20) is on the line y = 2x and therefore solutions exist. We check that the vector (10, 20) is on the line y = 2x by plugging the numbers x = 10, y = 20 into the equation y = 2x. As you can see 20 = 2 * 10. Which means the resulting vector (10,20) is on the line to which the vector space gets reduced, which means that there are solutions to the problem.For example, let's say that the matrix was the same but that the resulting vector was (10,50). In that case, there would be no solutions, because if we plug the numbers x = 10, y = 50 into the equation y = 2x we will get 50 = 2*10 which is false. Therefore the resulting vector (10, 50) doesn't belong to the line y = 2x. The resulting vector doesn’t belong to the line to which the vector space gets reduced (y=2x), which means that there are no values for x and y such that the vector (x, y) will give you the vector (10,50) after a matrix2 64 12is applied to it.You need a vector (x, y) such that when you apply the matrix (2, 6, 4, 12) to it you will get the vector (10, 50). There is no solution to this problem, there are no values for x and y such that vector (x, y) becomes vector (10, 50) after this matrix is applied to it.
How did the Big Bang happen? How has the universe evolved since then?
We have no idea how the Big Bang occurred, but we suspect that the Big Bang occurred because of quantum fluctuations in the nothingness, or possibly the multiverse. Unfortunately, because we cannot look outside our universe, we may never honestly know how the Big Bang started.Now, we will start with what we do know.The Big Bang SingularityThe Big Bang occurred from a singularity point with infinite mass and density in zero volume, which is predicted by general relativity for black holes. In the singularity, all known physics breaks down, including general relativity itself. A theory of everything will likely be able to describe the conditions of a singularity. Since we have yet to reach TOE, we do not know much about singularities. However, we know that the Big Bang came from this.Planck EpochThe Planck Epoch is the period before the universe was [math]10^{-43}[/math] seconds old. The universe was the size of Planck length, the temperature was above [math]10^{32}[/math] K, and the energy density was [math]10^{19}[/math] GeV. TOE is thought to apply during this period, but quantum gravity is the most dominant force through gravitons[1][1][1][1].Grand Unification EpochThis period is the universe up to [math]10^{-36}[/math] seconds old. The temperature is around [math]10^{29}[/math] K and the energy density at about [math]10^{16}[/math] GeV. Quantum gravity has already separated from the other forces, and the universe is ruled by GUT, the Grand Unified Theory[2][2][2][2].(Graph for reference)Inflationary/Electroweak EpochIn this time, the universe was about [math]10^{-32}[/math] seconds old, but the temperature and energy density went down several orders of magnitude. The temperature dropped to about [math]10^{22}[/math] K towards the end, while the energy density dropped to [math]10^9[/math] GeV. The reason for these large drops is because of inflation, which we will discuss later. Inflation caused the universe to expand extremely rapidly, outstripping the current dark energy-driven expansion we will also discuss later.The other important part of this period is the breakdown of GUT into the strong nuclear force and the electroweak force[3][3][3][3]. Gluons have separated from the photons, W+/-, and Z bosons. Since the temperature and energy density had dropped significantly, it was no longer hot enough to support a continued GUT universe.Quark EpochThe universe is about [math]10^{-12}[/math] to [math]10^{-6}[/math] seconds old. The temperature is around [math]10^{12}[/math] K and the energy density dropping to near 100 MeV, or about 0.1 GeV. This period is also very significant, as this is the time the Higgs field becomes essential. During this period, the electroweak force broke down, which is called spontaneous symmetry breaking[4][4][4][4]. This relates directly to the Higgs field, as the Higgs field gave mass to the W+/- and Z bosons of the weak nuclear force. As for electromagnetism, photons have remained massless.It is also during this time that the quark-gluon plasma exists[5][5][5][5][6][6][6][6]. Although the universe’s energy density has dropped quite a lot already, it is still too high for the formation of hadrons.Hadron EpochThe universe is about [math]10^{-6}[/math] to 1 second old. The temperature is about [math]10^{10}[/math] K, and the energy density is closing in on 1 MeV. It is now cool enough to form hadrons from the quark-gluon plasma[7][7][7][7][8][8][8][8]. During this period, the matter-antimatter asymmetry forms, otherwise known as baryon asymmetry[9][9][9][9], which we will discuss later.Neutrino DecouplingNeutrino decoupling occurs just at the end of the Hadron Epoch. Neutrinos began to interact with other particles very rarely. This is important, as we can observe the CNB, the Cosmic Neutrino Background, from this event. Unfortunately, since neutrinos rarely interact with matter because of decoupling, they are notoriously hard to detect[10][10][10][10]. However, we have managed to find the CNB[11][11][11][11][12][12][12][12][13][13][13][13][14][14][14][14].Lepton EpochThe universe is about 1 to 10 seconds old. The temperature is between [math]10^9[/math] and [math]10^{10}[/math] K, while the energy density is between 100 keV (0.1 MeV) to 1 MeV. This period is also significant, as it continues the matter-antimatter asymmetry in leptons[15][15][15][15][16][16][16][16][17][17][17][17][18][18][18][18]. Leptons, such as the electron, are in higher numbers than antileptons, possibly contributed by neutrino oscillations we will discuss later.NucleosynthesisThe universe is somewhere between 10 to 1000 seconds old. The temperature has dropped to near [math]10^7[/math] K, while the energy density drops from 100 keV to 1 keV. Because of the drop in temperature and energy density, atomic nuclei can form, but only up to beryllium. Most of the atomic nuclei had been in the form of hydrogen’s previously since single protons existed since the Hadron Epoch. However, now, helium nuclei could also form, with trace amounts of lithium and beryllium[19][19][19][19][20][20][20][20][21][21][21][21][22][22][22][22]. Hydrogen nuclei (protons) are still the most significant amount of baryonic matter.Photon EpochThis period goes along with Nucleosynthesis but lasts until the universe is about 380000 years old. The temperature has gone down from [math]10^9[/math] K to 4000 K. The energy density is about 0.4 eV at the end. The universe is a plasma of atomic nuclei and electrons, but mostly photons. Although the temperature and energy density has dropped, they are still too high for atoms to form. Because of this, the universe remains opaque.RecombinationThis time is one of the most critical events in the universe. The end of the Photon Epoch sees the universe finally become transparent. Electrons are now able to bind with nuclei, and the photons are released. This is where the CMB, the Cosmic Microwave Background, came[23][23][23][23].Dark AgesFor about the next 1 billion years, the universe is relatively dark. The CMB has redshifted out of the visible spectrum within 3 million years after Recombination. Atoms float freely throughout the universe[24][24][24][24].To 1687/Present DayThe first stars are said to have formed about 1 billion years after the Big Bang[25][25][25][25]. We have yet to confirm, but the JWST should be able to look back to this time in the universe. The first galaxies formed in the next few million years, which the last of we see here.Further stellar and galactic evolution, along with the universe’s, continues up to now. The universe also continued to decelerate its expansion[26][26][26][26].However, sometime between 7 to 9 billion years after the Big Bang, dark energy became a significant part of the universe. During this 2 billion year range, the universe’s expansion began accelerating, and it has continued to do so[27][27][27][27]. Because of this expansion, the temperature and energy density of the universe has dropped much faster. The current temperature is about 2.7 K, just like the CMB, while the CNB temperature is lower, at around 1.95 K.1687In 1687, Isaac Newton published the “Philosophiae Naturalis Principia Mathematica” (The Mathematical Principle of Natural Philosophy), which gave us a fundamental understanding of gravity[28][28][28][28]throughout the universe. Newton’s law of gravitation set the foundation of classical mechanics and remains one of the most important parts of physics because it showed a relationship between mass, distance, and the force of gravity. Unfortunately, his reasoning was slightly flawed since it ran on the fact that space and time were absolute in all frames of reference. Although Newton’s law was part of the success of finding the planets Uranus and Neptune, it failed to accurately predict the precession of Mercury's orbit, among many issues. However, because Newton’s law of gravitation proved successful in the majority of cases, few thought to replace it with a better theory until 1916.1801Young performed his double-slit experiment in 1801. With the result of the experiment, it was firmly proven that light was a wave and not a particle[29][29][29][29]as Newton had promoted previously. With this understanding, electromagnetism would be thought of as a wave for the rest of the 1800s, all based on the results of Young’s double-slit experiment. However, because there was no other testing beyond the double-slit experiment, physicists only believed that light was only a wave and was definitively not a particle, and this thinking would last until 1905.1860–1877From 1860 to 1877, James Clerk Maxwell and Ludwig Boltzmann derived a probability distribution called the Maxwell-Boltzmann distribution for idealized gases as thermodynamic equilibrium. Maxwell first derived it in 1860[30][30][30][30][31][31][31][31], but it was Boltzmann that derived the distribution under statistical thermodynamics, averaging the number of particles in each microstate and giving the ratio between the energy of a state to its temperature[32][32][32][32][33][33][33][33][34][34][34][34]. However, the probability distribution assumed no interaction between particles and that each one of their states could be considered independently of the states of other particles. Thus, future theories in statistical mechanics would be formulated under the observed deviations from Maxwell-Boltzmann statistics, specifically Fermi-Dirac statistics and Bose-Einstein statistics.1865In 1865, Maxwell published “A Dynamical Theory of the Electromagnetic Field,” which gave the equations necessary to describe electromagnetism[35][35][35][35][36][36][36][36]. It would remain as the best understanding of electromagnetism until 1928, although faith in Maxwell’s equations was deteriorating because of issues in predicting blackbody radiation.1879The Hall effect was discovered in 1879 by Edwin Hall, who was trying to explore the interactions between electric currents and magnetic fields, as had been established mathematically to exist by Maxwell less than 20 years earlier. Hall discovered that a transverse electric field is formed in a material with an electric current when placed in a perpendicular magnetic field[37][37][37][37][38][38][38][38]. This electric field results from the magnetic field exerting on the positively and negatively charged particles in the electric current. The perpendicular magnetic field displaces the charges at right angles to the magnetic field and electric charge, accumulating a charge on one side and the opposite charge on the other, creating a difference in potential. The sign of the voltage measured for the difference determines whether the current is positive or negative. Most metals have a negative Hall voltage because the electrons are carrying the current, but a few metals have a positive Hall voltage by the movement of a “hole,” which moves in the opposite direction of electrons trying to fill previous “holes.” The Hall voltage is also proportional to the electric charge, to the magnetic field, and to the behavior of the conductor being used. Different materials have different Hall coefficients, so the Hall voltage is not always the same and can change with temperature since the Hall coefficient does vary with temperature.The Hall effect proved that electric currents were carried by negative charges rather than positive charges. It offered an early clue to the existence of the electron, a precursor to Thomson’s discovery in 1897[39][39][39][39]. However, the quantum form of the Hall effect would turn out to be more complicated, from fractional values to spin currents.1887The Michelson-Morley experiment finally disproved the existence of aether in 1887[40][40][40][40][41][41][41][41], demonstrating that light had a constant speed in a vacuum, although it was hard to pin down an exact value for the velocity. Later experiments in the 20th century were able to obtain an exact value, while also disproving that particles can go faster than light[42][42][42][42][43][43][43][43][44][44][44][44][45][45][45][45].1900Max Planck finally solved blackbody radiation and the so-called ultraviolet catastrophe in “On the Theory of the Energy Distribution Law of the Normal Spectrum.” Through his law, Planck showed that energy was quantized, so radiation decreased along with wavelength in relation to temperature[46][46][46][46], rather than increase towards infinity that the Rayleigh-Jeans law had predicted. There were only specific values of frequency and wavelength that were possible, not a continuous set of frequencies and wavelengths.(The graph shows how the laws worked at 5800 Kelvin based on frequency. So, when the wavelength decreases, it means the frequency increases in this graph.)1905The year 1905 practically spelled the end of classical physics. Albert Einstein’s Annus Mirabilis papers, especially “On a Heuristic Viewpoint Concerning the Production and Transformation of Light” (photoelectric effect), “On the Electrodynamics of Moving Bodies” (special relativity), and “Does the Inertia of a Body Depend Upon Its Energy Content?” (mass-energy equivalence), nearly overthrew classical mechanics.The first paper[47][47][47][47][48][48][48][48](On a Heuristic Viewpoint Concerning the Production and Transformation of Light) described the photoelectric effect and showed that light was also a particle, a photon, not just a wave. With this, wave-particle duality was demonstrated for light.The third paper[49][49][49][49](On the Electrodynamics of Moving Bodies) described special relativity, showing that time was relative, that distances were relative, and that mass was relative to other frames of reference because the object’s velocity mattered. It also showed that the speed of light was constant, no matter the frame of reference, because it was a fundamental value that could be measured and calculated from different frames of reference.The fourth and final paper[50][50][50][50](Does the Inertia of a Body Depend Upon Its Energy Content?) showed a relationship between mass and energy. [math]E^2 = (pc)^2 [/math][math][/math][math]+ (mc^2)^2[/math] demonstrated that mass and energy could be converted into the other in relation to the speed of light.Because of Einstein, we realized that spacetime was relative to frames of reference and that there was no absolute. Also, there was a relationship between mass and energy. Finally, the property of wave-particle duality existed for light, and by extension, should apply to matter, although this was not yet recognized.1916In 1916, Einstein published his theory on general relativity in “The Foundation of the General Theory of Relativity.” Here, Einstein showed spacetime was also relative to gravity[51][51][51][51]. Gravity was an effect of mass on the structure of spacetime itself, so a frame of reference was relative to how spacetime was changed by a massive object. The closer an observer was to an object, the slower their time ran relative to an observer further away from the object. Black holes demonstrate this effect to an extreme. Closer to an event horizon, time runs slower relative to an observer further away. However, Einstein was fond of ridiculing predictions made by his theory, such as gravitational waves[52][52][52][52]and black holes[53][53][53][53].1919In 1919, Arthur Eddington proved general relativity correct by observing an eclipse on the island of Principe. Observing the May 1919 eclipse, Eddington saw that the stars near the sun were not in their correct positions, and were off by angles predicted by general relativity. Published in “A Determination of the Deflection of Light by the Sun's Gravitational Field, from Observations Made at the Total Eclipse of May 29, 1919,”[54][54][54][54][55][55][55][55]general relativity became the new theory of gravity, overthrowing Newton’s law of 232 years.(This was the Times headline following Eddington’s publication.)1924Since 1905, it had been proven that wave-particle duality existed for electromagnetic waves, but it was not until 1924 that Louis de Broglie proved the same for matter. In his doctoral thesis, “On the Theory of Quanta,” de Broglie showed that electrons, and by extension, all matter, could be observed in the form of waves if done correctly[56][56][56][56][57][57][57][57]. This was confirmed by experiments later in the decade, one by the diffraction of cathode rays[58][58][58][58]and the other by reflection off a nickel crystal[59][59][59][59]. The double-slit experiment proved this as well, once in 1961[60][60][60][60]and a second time in 2012[61][61][61][61]. de Broglie showed that wave-particle duality applied to all particles and that how one measured in an experiment changed what was observed.1924Later in the year, Satyendra Bose found that by applying an error from Maxwell-Boltzmann distribution made a prediction that correlated with experiments of photons. He realized that photons could be considered to have one variable for position and momentum together[62][62][62][62]because the Maxwell-Boltzmann distribution did not apply on quantum scales because it assumes different states for each particle at thermodynamic equilibrium. Einstein supported Bose’s paper on the statistics of photons[63][63][63][63][64][64][64][64], which eventually applied to all bosons. Under Bose-Einstein statistics, bosons of the same energy are utterly indistinguishable from each other because they can all occupy the same energy state without interacting with each other.1925In 1925, Wolfgang Pauli demonstrated that electrons could not be in the same quantum state at the same position and at the same time. In his paper, “On the Connexion between the Completion of Electron Groups in an Atom with the Complex Structure of Spectra,” Pauli showed that all four quantum numbers ([math]n, ℓ, m_ℓ, m_s[/math]) could not all be the same[65][65][65][65]. Three of the numbers could be the same for two electrons, but at least one could not be. This was specific to electrons alone in atomic orbitals, but Pauli extended the general rule to other spin-1/2 particles, which meant all fermions, with the spin-statistics theorem 15 years later[66][66][66][66].1925Later in the year, Werner Heisenberg developed matrix mechanics in his paper, “Quantum-Theoretical Re-interpretation of Kinematic and Mechanical Relations,” [67][67][67][67]and further developed by Max Born and Pascual Jordan in “On Quantum Mechanics.”[68][68][68][68]Heisenberg had been trying to predict spectral lines of hydrogen, but his idea of non-commutable observables led to Born realizing the use of matrices. “On Quantum Mechanics’ developed the rest of matrix mechanics in complement with “Quantum-Theoretical Re-interpretation of Kinematic and Mechanical Relations,” leading to Pauli deriving the hydrogen spectrum the following year in “About the hydrogen spectrum from the point of view of the new quantum mechanics.”[69][69][69][69]1926In 1926, Erwin Schrödinger published his equation in wave mechanics, which described the quantum-mechanical system like Heisenberg’s earlier matrix mechanics. His paper, “Quantization as an Eigenvalue Problem,” was developed throughout the year and eventually caused real numbers to be replaced with complex numbers throughout quantum mechanics[70][70][70][70][71][71][71][71][72][72][72][72]. Wave mechanics described the quantum-mechanical system much more cleanly than matrix mechanics but also gave the probabilistic wave function. Born interpreted the value of [math]ψ[/math] as the probability amplitude, and that [math]ψ^2[/math] was the probabilistic wave function[73][73][73][73][74][74][74][74]. The Born rule related Schrödinger’s equation to observations of the electron, although Schrödinger himself refused to accept this interpretation.1926In 1926, Enrico Fermi and Paul Dirac derived Fermi-Dirac statistics under the Pauli exclusion principle, to describe the behavior of the electron[75][75][75][75][76][76][76][76]. Experiments of the electron had also begun to wander away from predictions from Maxwell-Boltzmann distribution, because the model for metals considered all electrons equivalent to each other, and would contribute to the specific heat in orders of [math]k_B[/math]. Fermi-Dirac statistics solved the issue by demonstrating that the distribution of fermions could not have any of them in the same energy state.1927In 1927, Heisenberg developed the uncertainty principle to demonstrate that absolute certainty in measurements was not possible, using the equation [math]σx * σp >= \dfrac{ħ}{2}[/math] (sigma x * sigma p must be greater than or equal to the reduced Planck’s constant divided by two). In his paper, “About the descriptive content of quantum theoretical kinematics and mechanics,” Heisenberg showed that uncertainty in values depended on the other; if the value of σx or σp were smaller, then the value of σp or σx would be of a larger value[77][77][77][77]. The actual equation itself was developed later that year by Earle Kennard[78][78][78][78]. These two images show Heisenberg’s uncertainty principle. When the blue graphs (position) has a higher maximum, the red graphs (momentum) have a lower maximum. In the same way, when the red graphs have a higher maximum, the blue graphs have a lower maximum.1927Around the same time, Dirac began developing quantum electrodynamics. In his paper, “The Quantum Theory of the Emission and Absorption of Radiation,” Dirac explained that even at the ground state, the electromagnetic field was constantly oscillating at the minimum energy[79][79][79][79][80][80][80][80]. Using first-order and second-order perturbation theory, Dirac’s QED could account for absorption and emission of radiation from atoms, Compton scattering of photons, and resonance fluorescence. Further development by Pauli and Jordan[81][81][81][81][82][82][82][82], Fermi[83][83][83][83][84][84][84][84], and Heisenberg[85][85][85][85][86][86][86][86]bettered the theory, but at higher orders of perturbation theory, calculations ended in infinities, and there seemed to be no way to reconcile special relativity to quantum mechanics.1927About a month after both Heisenberg’s and Dirac’s papers, Georges Lemaître came up with his theory on the Big Bang. Lemaître believed that the universe was once a large atom in a tiny space, which exploded into all the atoms we see today[87][87][87][87][88][88][88][88]. Unfortunately, the theory showed that the universe was expanding, and this idea was mostly unacceptable to the physics community at the time, including Einstein. There was some exception to the mathematics behind Lemaître’s theory, but otherwise, it was wholly dismissed. Lemaître was vindicated shortly after, and he republished his work in 1931[89][89][89][89], but his version of the Big Bang idea was incorrect. The “primeval atom” did not work, but the basic concept of the Big Bang seemed possible, but lacked evidence during this time and needed further development.1928In 1928, Dirac published “The Quantum Theory of the Electron,” which successfully incorporated special relativity into quantum mechanics, while also describing all fermions of spin-1/2 with P-symmetry[90][90][90][90][91][91][91][91]. However, it also implied the existence of antimatter, and the absence of evidence for it seemed to be the issue Dirac had with his equation, but otherwise, the equation fitted perfectly with experimental observations. It was not until 1932 that the positron was confirmed by Carl David Anderson[92][92][92][92]and that there was definitive proof of the existence of antimatter. However, this would set off the debate about why there was a matter-antimatter asymmetry in the universe. Even with this, Dirac’s equation and prediction of antimatter remain two of the most significant elements of quantum mechanics and physics in general.19291929 was the year Lemaître was finally justified in his theory of an expanding universe, and it was done by no other than Edwin Hubble. Previously, Hubble had proven that the Milky Way was only one of many galaxies in the universe. Now, in “A Relation Between Distance and Radial Velocity Among Extra-Galactic Nebulae,” Hubble showed that these galaxies were receding away from us, with a few exceptions like the Andromeda Galaxy[93][93][93][93]. With this discovery, it proved Lemaître correct about the expansion of the universe, but it was somewhat inconclusive about the “primeval atom.”Following Hubble’s discovery, Einstein removed the cosmological constant out of general relativity, since he had previously placed it to accommodate a static universe. However, there was no definite evidence for Lemaître’s “primeval atom,” so it seemed that the universe had existed for an infinite time, and this was the consensus at the time. Fortunately, the basic idea of the Big Bang was more considered following Hubble’s discovery.On the other hand, Hubble’s measurements were somewhat mediocre. His value of the Hubble constant was about 500 km/s/Mpc, based on measuring Cepheid variables of 47 galaxies. Furthermore, distances to those Cepheid variables and their host galaxies were still debatable, which caused inadequate measurements. Better observations were necessary to measure the expansion of the universe accurately and, therefore, the Hubble constant.Even with this in mind, the Hubble constant was considered to be decreasing over time because the expansion was said to be decelerating. This was propagated by prominent figures in the field, such as Eddington[94][94][94][94][95][95][95][95]and Dirac[96][96][96][96], and eventually, Stephen Hawking[97][97][97][97]. There are some signs that the Hubble constant was a significantly larger value than expected going into 1998, but it still ranged from 40 km/s/Mpc to 100 km/s/Mpc, with no way to discern the value precisely[98][98][98][98][99][99][99][99][100][100][100][100][101][101][101][101][102][102][102][102][103][103][103][103][104][104][104][104][105][105][105][105][106][106][106][106].1931In 1931, Dirac developed the idea of the magnetic monopole. In electromagnetism, there was an asymmetry between the electric field and the magnetic field. Matter was either a positive or a negative charge if not neutral, in the electric field. In the magnetic field, however, matter had dipoles. There were no monopoles. This was the asymmetry in electromagnetism as well as quantum electrodynamics. Dirac came up with the theory in “Quantised Singularities in the Electromagnetic Field” to help explain magnetic monopoles as well as the lack of them[107][107][107][107][108][108][108][108]throughout the universe. He said that if magnetic monopoles existed, electric charge in the universe would be quantized. Electric charge in the universe was quantized, but that fact did not prove the existence of magnetic monopoles. There seemed to be experimental evidence for them in 1975[109][109][109][109]and 1982[110][110][110][110], but both were ruled inconclusive[111][111][111][111]. Later papers theorized that magnetic monopoles existed in the early universe[112][112][112][112][113][113][113][113][114][114][114][114][115][115][115][115]but came up with different results as to what happened to monopoles after that time.1933In 1933, Fritz Zwicky proposed the existence of dark matter. In “The Redshift of Extragalactic Nebulae,” Zwicky found that material in galaxies seemed to be rotating faster than calculated for a galaxy rotation curve, and this suggested that other material may be present while being unobservable[116][116][116][116][117][117][117][117]. Zwicky focused on the idea in a later paper, “On the Masses of Nebulae and of Clusters of Nebulae,” to demonstrate that some unobservable material had to exist to explain the speeds of stars in galaxies[118][118][118][118]. Unfortunately, Zwicky was not personally popular among his contemporaries, so his idea was generally ignored in the community. The idea of dark matter would not show up again until 1970.1935In 1935, Einstein began questioning quantum entanglement. Along with Boris Podolsky and Nathan Rosen, Einstein published “Can Quantum-Mechanical Description of Physical Reality Be Considered Complete?” to show that quantum entanglement allowed for a paradox violating Heisenberg’s uncertainty principle[119][119][119][119]. The thought experiment allowed for a value to be determined for both position and momentum, violating Heisenberg’s uncertainty principle, which states that it is impossible to know both. The paper concluded that the violation meant the reality described by wave mechanics was incomplete. Niels Bohr, who had argued with Einstein over quantum mechanics since 1927, rejected the EPR argument. Bohr stated that once a value was accurately measured for either position or momentum, it was impossible to measure the other[120][120][120][120].However, Einstein himself was not pleased with the description set in his paper, as Podolsky wrote most of the paper. Einstein believed in locality, and that quantum entanglement was incorrect because it violated causality[121][121][121][121][122][122][122][122]. He saw that entanglement caused information to be known faster than light, which should not be possible. The event of getting the information was outside the light cone of the measurement, which was the first event. Although the second event happened after the first, it happened before light could travel from the first event. Einstein believed that the second particle was unaffected by measurements of the first particle because of locality. The only option was the existence of local hidden variables to describe measured states of particles.Further development of hidden variables only worked in a nonlocal hidden variable theory, successfully made by David Bohm 17 years later. Like EPR, it was also deterministic, but it was based on nonlocality. de Broglie had first developed it as the pilot wave theory but later favored the Copenhagen interpretation, effectively abandoning his theory by 1927. However, Bohm developed it successfully to solve the measurement problem by stating the wave function only seems to collapse, but in reality, the wave packets are separated[123][123][123][123][124][124][124][124]. It showed that Heisenberg’s uncertainty principle made it impossible to get a definite value for any measurement.Unfortunately for both Einstein and Bohm, there was no way to prove the existence of hidden variables, local or nonlocal, until 1964.1935After the EPR paper, Schrödinger questioned the Copenhagen interpretation over quantum superpositions. In his thought experiment, a cat, a radioactive material, a Geiger counter, a hammer, and a container of poisonous gas were all in a box. If the Geiger counter detected a particle from the radioactive source, then the hammer would be released, breaking the container and releasing the gas, killing the cat. On the other hand, if the particle were not detected, the container would remain sealed, and the cat would still be alive. However, without observing inside the box, everything inside the box would be in a quantum superposition. Schrödinger specified on the cat as being in a superposition of being alive and dead[125][125][125][125][126][126][126][126]. He found this situation as ridiculous and that the cat must be one or another without observation. However, the experiment was correct that the cat was in a superposition, just like all the other materials inside the box. This was at the core of the measurement problem, where the wave function did not collapse until an observation occurred. Schrödinger stated through his paper that a superposition did not exist and that the wave function did not collapse just because an observer exists, but this was the truth.Other interpretations of Schrödinger’s cat, most notably, the many-worlds interpretation, had different responses to the thought experiment and the measurement problem in general. Under the many-worlds interpretation, the measurement problem did not exist. It stated that all states would exist when an observation occurs, but these states cannot interact with each other because of decoherence. For Schrödinger’s cat, both states of an alive cat and a dead cat exist, but neither state can interact with the other.Under Bohm’s interpretation, Schrödinger’s cat is in a superposition since the wave function never collapses. However, measuring one of the materials inside the box, without measuring the other materials or the cat, then it becomes clear what the state of the cat is. Quantum entanglement forces a measurement of the cat’s state from measuring the state of any other material inside the box.1948Although Lemaître’s “primeval atom” was incorrect, the idea of the Big Bang was reintroduced in “The Origin of Chemical Elements,” authored by Ralph Alpher, Hans Bethe, and George Gamow. The paper explained how all the elements were formed in the Big Bang, leaving vast amounts of hydrogen and helium and more trace amounts of the following elements[127][127][127][127]. The paper stated that the elements formed when nuclei combined with more neutrons, some of which decayed into protons, forming new elements. The calculations fit with observations of hydrogen, helium, and their isotopes throughout the universe but failed due to the mass gap from five to eight atomic units. There were no stable nuclei in this range, therefore hindering further production of elements beyond helium. Due to this flaw, the successive capture theory was disregarded for elements beyond helium, but the paper was accepted partially for correctly predicting the amount of each isotope for hydrogen and helium.However, the mass gap between helium and carbon was a significant issue that had to be resolved. Further development into nucleosynthesis was necessary to explain the existence of higher elements.1950Since 1930, issues with quantum electrodynamics had led many to believe that special relativity and quantum mechanics were incompatible. Robert Oppenheimer first pointed out that the theory worked out well only in first-order perturbation theory[128][128][128][128], proven further by studies in 1937[129][129][129][129]and 1939[130][130][130][130]. Improvements in measurements found the Lamb shift and the magnetic moment of the electron[131][131][131][131][132][132][132][132], which could not be explained. However, in 1947, Bethe hinted a way out by renormalization, giving finite results that matched experiments[133][133][133][133].Including Bethe’s renormalization, eleven other papers were able to formulate QED at any order of perturbation theory. In 1946, Shin'ichirō Tomonaga was able to reincorporate special relativity in an invariant formulation, stating that previous works were too closely related to non-relativistic mechanics[134][134][134][134]. Two years later, Julian Schwinger developed on Lorentz covariance for the formulation[135][135][135][135][136][136][136][136], then applied it to the vacuum[137][137][137][137]and the energies of the electron and photon[138][138][138][138].Around the time Schwinger was developing the applications of his covariant formulation, Freeman Dyson and Richard Feynman were also deriving formulations. Feynman had already created the famed Feynman diagrams, which Dyson used to compare with Tomonaga’s and Schwinger’s formulations[139][139][139][139]. Dyson then used the scattering matrix and renormalization for higher orders of perturbation theory[140][140][140][140]. Following this, Feynman published his mathematical formulation that was based on his diagrams[141][141][141][141][142][142][142][142][143][143][143][143], rather than the functions used by Tomonaga and Schwinger.It was now finally possible to have covariant formulations that were finite at any given order of perturbation theory, and quantum electrodynamics became the model for quantum chromodynamics and the basis for the electroweak theory.1953–1956While quantum electrodynamics had finished, quantum chromodynamics had just started. Experiments continued to find more hadrons, and it became harder to believe that they were all fundamental particles.Heisenberg had introduced the concept of isospin back in 1932 for the proton and neutron[144][144][144][144], and Eugene Wigner introduced the term isospin to explain how it was related to spin[145][145][145][145]in classical mechanics. The isospin value and the charge value were how hadrons were classified but it did not justify the fact that too many hadrons existed. Furthermore, particles such as kaons and hyperons decayed slower than expected. This pointed to a possible new value that was conserved in the creation of kaons and hyperons but was not in their decays.This new value was strangeness. Kazuhiko Nishijima developed strangeness in 1953, although he called it η-charge after the η meson[146][146][146][146]. In 1955, Nishijima developed the equation, [math]Q = I [/math][math][/math][math]+ \dfrac{B [/math][math][/math][math]+ η}{2}[/math], where Q was the electric charge, I was the isospin, B was the baryon number, η was the η-charge[147][147][147][147]. Murray Gell-Mann independently derived the same equation in 1956, also creating the new value of strangeness[148][148][148][148].However, this strangeness value was not understood. There was no underlying theory that could explain this third value, but it was definite that elementary particles made up the hadrons.1957In 1957, it was finally understood how elements were formed beyond helium and past the mass gap between five and eight atomic units. In “Synthesis of the Elements in Stars,” the Burbidge couple, William Fowler, and Fred Hoyle explained that stellar nucleosynthesis created elements beyond lithium and elements beyond iron by the capture of free neutrons[149][149][149][149].However, the theory was incorrect, as it failed to explain elements from silicon to nickel. Hoyle believed that those elements formed in supernova nucleosynthesis three years prior[150][150][150][150], but it was also incorrect. Stellar nucleosynthesis was based on carbon-burning, oxygen-burning, and silicon-burning processes to create the elements up to iron, but none were mentioned in the paper. Furthermore, the elements beyond iron were created in supernova nucleosynthesis, not stellar nucleosynthesis.Previously, Hoyle had already explained the formation of elements up to iron with the previous processes in mind back in 1946[151][151][151][151]. This work was based on Bethe’s, who had already explained the forming of elements up to oxygen[152][152][152][152].Following B2FH paper, the theory of stellar nucleosynthesis was improved by Alastair Cameron[153][153][153][153]and Donald Clayton[154][154][154][154][155][155][155][155][156][156][156][156][157][157][157][157]over the next decade.1959In 1959, Sheldon Glashow and Abdus Salam came up with the electroweak theory. The theory was based on the violation of P-symmetry in the Wu experiment and an earlier experiment. The experiment previous to the Wu experiment had observed a parity violation in the decay of kaons. One kaon decayed into two pions while the other kaon decayed into three pions, but they were the same kaon. It was assumed that weak interactions would follow P-symmetry, but the decay of the exact same kaon in two different processes became the τ–θ puzzle[158][158][158][158]. The following Wu experiment was meant to confirm whether P-symmetry existed in weak interactions based on the first experiment. The result was a violation, which meant there was handedness in weak interactions[159][159][159][159].After the Wu experiment, Glashow combined a chiral symmetry and an achiral symmetry into one new symmetry, but this could not be renormalized as quantum electrodynamics had been. It predicted the existence of a Z boson, but there was no experimental evidence of it, so the theory was little recognized[160][160][160][160]. Salam also came up with the same conclusion[161][161][161][161], so the electroweak theory had little support. However, it also introduced spontaneous symmetry breaking, which had to be proven along with the existence of the vector bosons. Unfortunately, the electroweak theory still needed work.1961–1965In 1961, Gell-Mann sorted the hadrons into the eightfold way, which helped recognize similarities[162][162][162][162]. Using values of isospin, charge, and strangeness, it was now possible to see an underlying and fundamental structure to hadrons. The fundamental structure remained unknown, but it had to conserve the properties of isospin, charge, and strangeness.This was achieved two years later by Gell-Mann and, independently, George Zweig. Gell-Mann theorized the existence of three quarks to explain the properties of the hadrons, the up quark, the down quark, and the strange quark[163][163][163][163]. Zweig, calling them aces, theorized four particles under the SU(3) model over Gell-Mann’s eightfold way[164][164][164][164][165][165][165][165]. Quarks were considered fundamental particles along with leptons. With an underlying structure for hadrons, there was no reason to develop the theory any further.Two years later, Boris Struminsky suggested that another degree of freedom existed for quarks[166][166][166][166]. The recently discovered Ω− baryon was composed of three strange quarks of parallel spin, therefore violating the Pauli exclusion principle unless another degree of freedom existed. Struminsky added in a footnote, “Three identical quarks cannot form an antisymmetric S-state. In order to realize an antisymmetric orbital S-state, it is necessary for the quark to have an additional quantum number.” This was followed by another paper that discussed the possible new degree of freedom[167][167][167][167].The situation was verified by the Δ++ baryon, which was three up quarks of parallel spin. By this point, a paper by Oscar Greenberg had hinted at an additional SU(3) gauge degree of freedom[168][168][168][168]. A later paper by Moo-Young Han and Yoichiro Nambu furthered that the quarks interact by eight vector gauge bosons within the hadrons[169][169][169][169].Unfortunately for Gell-Mann and the other physicists involved, there was no experimental evidence for free quarks, so the theory was stunted at the moment. Gell-Mann’s only explanation was that quarks were confined in hadrons but also that the theory was incomplete.1964While quarks were being theorized, the electroweak theory was given new consideration. Glashow and Salam had introduced spontaneous symmetry breaking in their electroweak theories, but there was no known process to give mass to the W+/- and Z bosons. The underlying mechanism was proposed in 1962, but there was no relativistic theory to explain the mechanism[170][170][170][170]. However, in 1964, three independent groups were able to explain spontaneous symmetry breaking, and mass generation in general, without technically violating gauge symmetry[171][171][171][171][172][172][172][172][173][173][173][173]. Each of the PRL symmetry breaking papers described a scalar field that developed a vacuum expectation value at a critical temperature. The field’s symmetry is broken by tachyon condensation, where the field lowers its energy by spontaneous creation of particles. This process would give the W+/- and Z bosons mass, which was the spontaneous symmetry breaking.The theory also allowed a way to avoid Goldstone bosons. Under Goldstone’s theorem, the ground state of the vector bosons would not be invariant, so massless scalar bosons would be necessary for the symmetry breaking to occur[174][174][174][174]. The PRL symmetry breaking papers helped avoid this under a gauge-invariant model.Unfortunately, there was no way to prove the existence of any scalar boson and field. Because the theory required large values, colliders and accelerators could not detect the theorized boson. The theory was consistent with Glashow and Salam’s form of the electroweak theory, but there was no way to prove either theory correct. It would be necessary to detect the W+/- and Z bosons before any more massive particle, in theory, could also be detected.1964Twenty-nine years prior, Einstein believed that in locality over quantum entanglement and explained that local hidden variables existed to create the results of measurements predicted under entanglement.Under locality, he believed that causality would not be violated, unlike under entanglement. The hidden variables could produce results of the second particle without any relation with the measured first particle with locality. The particles were related under entanglement, so measuring one particle would then yield the result of the second particle, therefore, based on Einstein’s belief, violating causality.In 1952, Bohm had successfully created a nonlocal hidden variable theory. With Bohm’s interpretation, particles were already determined to be observed in a particular state, no matter if a measurement occurred. Measuring the first particle instantaneously changed the state of the second particle, but under Bohm’s interpretation, those states were determined before the measurement of the first particle. The first particle would have some state no matter if observation occurred, which would also apply to the second particle. Hidden variables existed to determine those states, but they were not local to each particle but throughout the universe. Hidden variables existed in the whole universe because the system is the universe under Bohm’s interpretation.Bohm’s interpretation inspired Bell to disprove the existence of hidden variables, local or nonlocal. Before the third PRL symmetry breaking paper, Bell published “On the Einstein Podolsky Rosen Paradox” to disprove the existence of local hidden variables. He stated that independent measurements of two particles would be constrained on the results if hidden variables exist locally[175][175][175][175]. This constraint is the Bell’s inequality, and if EPR is correct, measurements should follow the inequality. If quantum entanglement is correct, then the inequality should be violated.However, Gerard ’t Hooft believed that Bell’s theorem had a loophole in superdeterminism, in which local hidden variable theories could arise[176][176][176][176][177][177][177][177]. However, Bell saw superdeterminism as implausible because a hidden variable or multiple hidden variables could not be sensitive to small effects as much as a deterministic random number generator used in the experiment[178][178][178][178].Predictions made by quantum mechanics do violate Bell’s inequality, demonstrating that hidden variable theories, such as EPR, cannot be local. However, it does not rule out nonlocal hidden variable theories, such as Bohm’s interpretation.Over the next 65 years, Bell’s inequality was violated by experiments, proving local hidden variables theories impossible, and some nonlocal nonBohmian hidden variable theories. The first[179][179][179][179]Bell test used the CH74 inequality, successfully violating Bell’s inequality in 1972. The second experiment used three separate tests, the first and third as CH74, and the second as CHSH[180][180][180][180][181][181][181][181]. In 1998, experiments began to measure across more considerable distances[182][182][182][182][183][183][183][183]and made improvements since the second experiment[184][184][184][184]. In 2000, experiments began using at least three particles[185][185][185][185]and in 2001, closed the detection loophole[186][186][186][186][187][187][187][187].An experiment in 2007 was the first to rule out nonlocal hidden variable theories, specifically those of Anthony Leggett[188][188][188][188][189][189][189][189][190][190][190][190]. In 2008, a test was done with a separation of 18 km to allow for completed measurements before any transfer of information between detectors[191][191][191][191][192][192][192][192]. In 2009, an experiment became the first to use solid-state qubits but under the locality loophole[193][193][193][193]that weakened results.Three more experiments in 2013 and 2014 closed all loopholes for the use of photons[194][194][194][194][195][195][195][195][196][196][196][196][197][197][197][197][198][198][198][198][199][199][199][199], culminating in three independent experiments where all loopholes were closed simultaneously[200][200][200][200][201][201][201][201][202][202][202][202][203][203][203][203].This was followed by a 2016 experiment using 480 atoms of a Bose-Einstein condensate, proving Bell’s theorem macroscopically, although the loopholes were open[204][204][204][204]. The following year, Bell’s theorem was tested cosmically via light from a star 600 light-years away[205][205][205][205]while another experiment tested spin states of two atoms separated 398 m while closing all three loopholes[206][206][206][206].In 2018, an experiment was the first to use the free will of 100,000 people under Bell’s theorem[207][207][207][207][208][208][208][208][209][209][209][209], which closed the “freedom of choice” loophole. An experiment later that year used quasars 7.8 billion light-years away, which was a significant part of the timeframe put by superdeterminism[210][210][210][210].Through all these experiments, Bell’s inequality has always been violated without exception. It has fundamentally proven that all local and some nonlocal hidden variable theories cannot predict states of particles as well as quantum mechanics does. The only hidden variable theory that still stands is Bohm’s interpretation from 1952, still successfully predicting as well as quantum mechanics through each experiment. Experiments testing Bell’s theorem in the future will have to find some way to prove Bohm’s interpretation incorrect, therefore ensuring overall that all nonlocal hidden variable theories cannot predict as well as quantum mechanics.1965While most of the field had been concerned with quantum chromodynamics, spontaneous symmetry breaking, and Bell’s theorem, Arno Penzias and Robert Wilson discovered the cosmic microwave background. In 1964, they detected a uniform noise spread throughout the sky but could not understand the noise. Discussing this information with Robert Dicke, it was realized that the noise was the cosmic microwave background predicted by Gamow 17 years earlier[211][211][211][211]. Dicke, along with Jim Peebles, Peter Roll, and David Wilkinson, had rederived Gamow’s theory, and proof of the CMB from Penzias and Wilson established that the Big Bang theory was correct[212][212][212][212].This disputed Hoyle’s steady-state model[213][213][213][213]that the universe was expanding eternally with new matter and energy forming to keep the mass-energy density of the universe constant. However, the discovery of the CMB fatally undermined the steady-state model. The model theorized that the CMB was light from stars and scattered by galactic dust, but the CMB was isotropic, and not polarized as would be expected by scattering.Hoyle continued to deny the Big Bang theory and developed the quasi-steady-state model in 1993. In the new model, Hoyle tried to explain, accounting for observations that the original model failed to explain. It explained that the universe had “little bangs” of creation over time to account for observations[214][214][214][214][215][215][215][215][216][216][216][216][217][217][217][217][218][218][218][218]and eventually, the accelerating expansion of the universe[219][219][219][219]after Hoyle’s death. By 1994, cosmologists, such as Edward Wright, criticized the QSS model and its predictions[220][220][220][220]while Hoyle tried to defend his theory[221][221][221][221]pointlessly. Even after Hoyle’s death, cosmologists have continued to find flaws with QSS[222][222][222][222]without replies.While Hoyle continued to defend his models, cosmologists began measuring the cosmic microwave background. After Penzias and Wilson’s detection, Rainer Sachs and Arthur Wolfe predicted fluctuations in the CMB from variations in the gravitational field, the Sachs-Wolfe effect[223][223][223][223], which predicted that gravitational redshift would affect the CMB photons. Martin Rees and Dennis Sciama also predicted fluctuations, but by time-dependent potential wells[224][224][224][224], where photons would need to gain energy to leave the potential well. The Sunyaev-Zel’dovich effect, predicted by Rashid Sunyaev and Yakov Zel’dovich, showed that inverse Compton scattering would occur between high-energy electrons in galactic clusters and the CMB photons[225][225][225][225], which was successfully proven in 1983[226][226][226][226]. By 1994, COBE had measured the CMB to have a black body curve similar to a real black body[227][227][227][227]but anisotropic on smaller scales[228][228][228][228]. The polarization of the CMB by the first atoms in the universe was predicted by the current ΛCDM cosmological model and was proven by DASI (Degree Angular Scale Interferometer) in 2002[229][229][229][229][230][230][230][230]. The polarization was confirmed to be E-mode polarization two years later by CBI (Cosmic Background Imager)[231][231][231][231][232][232][232][232]. WMAP improved measurements during its operation, establishing the ΛCDM model as consistent with CMB observations. The Planck Telescope has improved further on CMB observations but has turned attention away from the CMB itself to measuring the expansion of the universe based on the redshift of the cosmic microwave background.1967In 1967, Steven Weinberg formulated a renormalizable electroweak theory while reviewing spontaneous symmetry breaking. He had found symmetries that predicted a massless and neutral gauge boson, the photon. When Weinberg realized that the symmetries were the electroweak theory, he was able to predict the masses of the W+/- and Z bosons, leading to a working electroweak theory describing both electromagnetic and weak interactions[233][233][233][233]. However, because of the significant masses of all three bosons, it became necessary to upgrade particle accelerators and detectors to higher energies to prove the theory. The main reason was that the Z boson behaves just like a photon but is only detectable at higher energies because of its larger mass compared to the W+/- bosons.The first significant proof for the electroweak theory came in 1973 by the Gargamelle collaboration. From December 1972 to March 1973, the bubble chamber detected leptonic events, leading to neutral currents by neutrinos[234][234][234][234][235][235][235][235]. This was in support of the electroweak theory, as neutral currents in neutrino scattering were evidence of the Z boson.The W+/- and Z bosons were finally detected in 1983 via the UA1 and UA2 experiments[236][236][236][236][237][237][237][237][238][238][238][238]. Both UA1 and UA2 tried to find the W+/- bosons through its leptonic decay, finding ten candidate events in total. However, by theory, the Z boson would be more challenging to detect because of its larger mass, so the experiments required more energy in order for UA1 and UA2 to detect decay processes from the Z boson per the electroweak theory.1967Following Weinberg’s paper on renormalizable electroweak theory, Werner Israel gave the first version of the no-hair theorem based on the Schwarzschild metric[239][239][239][239]. The no-hair theorem was eventually applied to charged[240][240][240][240]and spinning[241][241][241][241]black holes. Under the no-hair theorem, all information that passed the event horizon of a black hole was lost to external observers. Observers within the event horizon would be able to access the information, but observers beyond the event horizon would have no process to obtain the information. In 1973, John Archibald Wheeler explained the no-hair theorem in “Gravitation.”[242][242][242][242]All black hole solutions to Einstein’s field equations and Maxwell’s equations in general relativity demonstrate that only mass, electric charge, and angular momentum can be observed externally. All information beyond these three parameters cannot be observed without passing the event horizon.However, because the information is lost to the universe beyond the black hole, it violates the no-hiding theorem of quantum mechanics. When information is lost from decoherence of a system, it cannot remain between the system and the environment of the system, because of the linearity and unitarity of quantum mechanics. Information must appear in another form that can be accessible to any observer within the universe to comply with this theorem.Under the no-hair theorem, however, information seems to be lost to the rest of the universe. Observers cannot access the information beyond the event horizon. They can obtain the information once they cross the event horizon, but they cannot communicate that information to the rest of the universe; therefore, the information remains lost.The fundamental example of the no-hair theorem compares two black holes. Both black holes have the same mass, electric charge, and angular momentum. The first black hole was formed out of matter while the second black hole was formed from antimatter. The no-hair theorem states that the two black holes are utterly indistinguishable for any observer beyond the event horizon. Because only mass, electric charge, and angular momentum are conserved, all other values, such as baryon number, lepton number, and quark number, are information lost from the universe. No observer beyond the event horizon can obtain that information because those values are not considered to be observables under all black holes solutions. By crossing an event horizon, an observer can obtain information about both black holes and conclude that one black hole was created out of matter and the other of antimatter, but that information cannot be communicated past the event horizon. The information remains lost to the universe because the observer is also lost to the universe. Once past the event horizon, the observer cannot return to the rest of the universe and communicate information on the two black holes.The no-hair theorem still applies to a third black hole, a kugelblitz black hole. A kugelblitz is created when energy is concentrated enough to form an event horizon and was first mentioned by Wheeler in 1955[243][243][243][243]. Since energy can be converted to mass via mass-energy equivalence given to us by Einstein, the kugelblitz has a mass. If we assume that the kugelblitz has a mass, electric charge, and angular momentum equal to that of the first two black holes, then all three black holes are entirely indistinguishable from each other to all observers beyond their event horizons. If the observer crossed one of the event horizons, the observer would know whether the black hole was created by matter, antimatter, or energy. However, unlike the original model, where the observer could say how the other black hole was created, this model does not allow the observer to know how the other black holes were created. Since the observer can only know about the black hole in which the event horizon was crossed, there is no method to discern between the other two black holes about what they created from out of the other two possibilities remaining.Assuming the observer obtains enough information within one black hole, the observer says that the black hole was made of matter. As in the original example, that information cannot be communicated to the rest of the universe when applying the no-hair theorem. However, considering the observer alone, there are only two options for the other two black holes. One must be made of antimatter and the other of energy. However, the observer can never know once past the event horizon of the given black hole. The best the observer can know is the mass, electric charge, and angular momentum of the other two black holes from previously made measurements.This same principle applies within the antimatter black hole and the kugelblitz. It is impossible to know between the other two black holes what they were before collapsing. However, in both the original model and the three-black-holes model, the observer can still never transmit information to the rest of the universe. The information remains within each black hole no matter the model when using the no-hair theorem, and the information will always be lost.Because the no-hair theorem came into direct conflict with the no-hiding theorem concerning the loss of information from the universe, it became necessary to find a way to preserve information and make the information accessible to observations. The most apparent option would turn out to be black hole thermodynamics.1970In 1970, Vera Rubin published “Rotation of the Andromeda Nebula from a Spectroscopic Survey of Emission Regions,” which theorized the existence of dark matter in galaxies[244][244][244][244]. Dark matter had been previously theorized by Zwicky in 1933 but had been forgotten because there was little experimental data on galaxy rotation curves to support the idea. Rubin’s galaxy rotation curve on the Andromeda Galaxy proved that unobservable matter was causing the galaxy’s rotation curve to be flatter than expected, as later measurements also confirmed[245][245][245][245]. Stars near the center were expected to have increasing velocity further away, but then eventually decrease as distance increased. However, observations showed that, while the rotation curve was correct close to the center, the actual rotation curve showed that stars had similar velocities in the rest of the graph. The curve flattened, signaling the existence of unobservable matter, causing stars to have faster velocities than expected. It was not out of the question that the observation could be specific for the Andromeda Galaxy, and that other galaxies would have expected galaxy rotation curves. However, Rubin did measurements of rotation curves in the Milky Way and observed a similar rotation curve as Andromeda’s[246][246][246][246].Later measurements found similar rotation curves for other galaxies[247][247][247][247][248][248][248][248][249][249][249][249][250][250][250][250][251][251][251][251][252][252][252][252], proving the existence of dark matter in galaxies. It was indisputable that some type of matter surrounded galaxies, but what type of matter was not clear. Measuring with light, partially gave insight because it proved that dark matter was not intergalactic gas, but also proved that dark matter had an observable gravitational effect. It was possible to simulate where the dark matter was, such as in the Bullet Cluster, which had some controversy over the ΛCDM model[253][253][253][253][254][254][254][254].1973In 1973, Gell-Mann theorized the color force to explain the Ω− and Δ++ baryons. Previously, Struminsky had mentioned that the Ω− baryon should not exist when applying the Pauli exclusion principle unless another degree of freedom existed. Greenberg had theorized a SU(3) degree of freedom in 1964, as did Han and Nambu the following year. However, because Gell-Mann’s explanation of quarks could not be verified, both papers went unnoticed. In 1969, Feynman proposed the parton model to explain that quarks were distributed in position and momentum, while the diffusion of momentum could explain diffractive scattering in collisions[255][255][255][255]. Gell-Mann believed that the charge of quarks could be localized in spacetime, but that the quarks might not be able to because spacetime could break down as shown in S-matrix theory. However, the S-matrix theory approach was abandoned when deep inelastic scattering was observed when colliding electrons into atomic nuclei[256][256][256][256][257][257][257][257].However, the color force was the most successful explanation for quarks to follow the Pauli exclusion principle using the Yang-Mills theory. The Yang-Mills theory was a general gauge theory formulated in 1954 and was based on the group theory of SU(n)[258][258][258][258]. Gell-Mann used the Yang-Mills theory to explain the color force, where the carrier particles, the gluons, could radiate more carrier particles[259][259][259][259]. Quantum electrodynamics was also based on the Yang-Mills theory, but photons did not radiate more photons, while in quantum chromodynamics, gluons did radiate more gluons. With the success of the color force, quantum chromodynamics was complete.Previously, however, it had been necessary to deal with the issue of confinement. Gell-Mann introduced the concept of confinement when experiments failed to see free quarks, but the observation could be explained with asymptotic freedom. Under asymptotic freedom, quarks and gluons would remain confined at lower energies and increasing distance. However, interactions between the particles would weaken as energies increased, and distance decreased. This occurred in the early universe during the Quark Epoch when the temperature and energy density of the universe was too high to allow hadrons to form. Because of asymptotic freedom, the quarks and gluons were not confined during this period, creating a quark-gluon plasma.The theory of asymptotic freedom was first made in June by David Gross and Frank Wilczek[260][260][260][260], followed independently by David Politzer[261][261][261][261]. The calculations showed that SU(3) was asymptotically free if there were 16 or fewer flavors of quarks. The theory demonstrated that no experiment during the period could have observed free quarks because of confinement, but it was possible to make accurate predictions under perturbation theory.However, because the strong nuclear force increases with distance, it is impossible to remove quarks and gluons from hadrons unless energy increases or distance decreases. This is verified by lattice quantum chromodynamics[262][262][262][262][263][263][263][263][264][264][264][264]but has not been mathematically confirmed. It remains necessary to prove a quantum Yang-Mills theory and the mass gap caused by confinement[265][265][265][265].1974–1975In 1974, Stephen Hawking theorized Hawking radiation for black holes[266][266][266][266]to follow along with the laws of thermodynamics. The second law required black holes to have entropy, and that they must increase in entropy more than the loss of entropy of matter-energy crossing the event horizon. Previously, Jacob Bekenstein formulated that the black hole entropy was proportional to the surface area of the event horizon divided by the Planck area. Hawking showed that black holes radiate to their temperature in the formula [math]S_{bh} = \dfrac{k_b×A}{4l_{p}^2}[/math], where [math]S_{bh}[/math] was the radiation, [math]k_b[/math] was the Boltzmann constant, [math]A[/math] was the surface area, and [math]l_p[/math] was the Planck length[267][267][267][267]. Using this formula, it could be predicted how much a black hole would radiate at a specific surface area.Hawking radiation demonstrated that black holes could radiate energy until they entirely evaporated. Under the theory, virtual particles at the event horizon become real because of the gravity of the black hole. One of the particles leaves the vicinity of the black hole at near the speed of light, while the other goes into the theorized singularity. The process gives the black hole negative energy, which forces the black hole to lose mass through mass-energy equivalence. Thus, black holes have a finite lifetime, which is the cube of the original mass given[268][268][268][268], as calculated by Don Page. However, based on the formulation, the lifetimes of all known black holes are significantly longer than the universe’s current age. Only Schwarzchild black holes of about [math]10^{14}[/math] g in the early universe would have evaporated by now, and a smaller mass for Kerr and Kerr-Newman black holes. Better measurements have a value of (5+-0.04)[math]×10^{14}[/math] g for Schwarzchild black holes[269][269][269][269].However, this was not the issue with the theory. Hawking believed that the no-hair theorem was correct, so the theory stated that all radiation was independent of information entering the black hole, which was a violation of the no-hiding theorem. If this occurred, once a black hole had evaporated, all information that had entered would be lost from the universe and be clearly in violation of quantum mechanics. The Bekenstein bound only gave an upper limit on the amount of entropy and information in a finite region of spacetime[270][270][270][270], but there is no lower limit. It is possible for a region of spacetime to not contain any information.There are several solutions to Hawking radiation while applying the no-hair theorem. The first is that information is completely lost from the universe, but it directly violates unitarity in quantum mechanics[271][271][271][271]. The second allows for information to “leak” during evaporation but deviates from general relativity and semiclassical gravity. The third states that all information escapes the black hole just before the black hole has totally evaporated, which would involve quantum gravity, but violate the Bekenstein bound for containing an arbitrary amount of information in a finite space. The next solution is that information is stored in a Planck-sized or large remnant, which would not require information to escape, but create infinite internal states at Planck length, or stopping Hawking radiation before Planck size, violating semiclassical gravity. The sixth solution states that the information is stored in a separate universe but currently cannot be tested currently. The last solution states information is correlated between the past and the future, which works under semiclassical gravity without dependence on quantum gravity, but directly violates time asymmetry.A solution to Hawking radiation without the no-hair theorem is “hairy” black holes. Because there is no definite mathematical proof of a general no-hair theorem, “hairy” black holes may be possible. Under this idea, information would be encoded on the event horizon of the black hole, forming “hair” on the event horizon. While violating the no-hair theorem, the no-hiding theorem remains. Information is conserved by the black hole and does not violate the Bekenstein bound. However, LIGO observations found evidence supporting the no-hair theorem in 2016, which discredited the “hairy” black holes idea. Hawking did calculations for “soft hair” that gave black holes more degrees of freedom while also existing at lower energies[272][272][272][272][273][273][273][273]that the no-hair theorem could not have calculated[274][274][274][274]. This would allow information to exist on the event horizon without being observed due to the low energy state it existed in.An issue with Hawking radiation is the trans-Planckian problem. Because the particles measured have a finite frequency, they can be traced back to the event horizon to have an infinite frequency, and therefore, a trans-Planckian wavelength. Lengths are considered meaningless when less than Planck length, but the particles must exist if they can be measured. The Hawking effect describes field modes that change frequency relative to spacetime outside the event horizon. The frequency of the particles can be regular if the field modes are extended into a past that observers cannot reach, so the particles can be sourced back to the point the black hole was formed in spacetime. Fluctuations at that point contain all the particles, and the field modes are redshifted into a trans-Planckian wavelength. Unfortunately, many find Hawking’s calculations ineffectual because physics is unknown at trans-Planckian scales[275][275][275][275][276][276][276][276][277][277][277][277][278][278][278][278].However, the most severe issue with Hawking radiation is quantum entanglement. Under quantum field theory, the particles in Hawking radiation are entangled. Assuming that the black hole has formed in a finite time in the past, that it will fully evaporate at a finite time in the future, and that half the possible information that can be emitted has been at time t, the particle leaving the black hole is entangled to all the information that has been emitted. Page and Leonard Susskind stated that a black hole with a finite past and a finite future would emit only a finite amount of information in its Hawking radiation[279][279][279][279][280][280][280][280][281][281][281][281][282][282][282][282]across its lifetime, and once at time t, when more than half of that information has been emitted, the particle leaving the black hole must be entangled with all that emitted information. However, this creates a paradox because that particle cannot be entangled in two independent systems at the same time.One controversial way to solve the “monogamy of entanglement” is the AMPS firewall. Under the firewall theory, the entanglement between the emitted particle and its pair is broken, but doing so releases energy at the event horizon, creating the firewall[283][283][283][283][284][284][284][284][285][285][285][285][286][286][286][286], which is fed further by the disintegration of matter-energy crossing the event horizon. However, the AMPS firewall violates Einstein’s equivalence principle, which states that free-falling in a gravitational field will always be indistinguishable to floating in a vacuum. The authors created a second theory without a firewall, but it violated quantum mechanics. The only other option available was to say the emitted particle was not entangled with all previously emitted information, but that would violate unitarity[287][287][287][287]. There had been no successful resolution to the AMPS firewall paradox since its inception in 2012, because it was only possible to do so by violating either the equivalence principle, quantum mechanics, or unitarity. However, this may have changed.1980In 1975, Tsuneya Ando, Yukio Matsumoto, and Yasutada Uemura predicted the integer quantization of the Hall conductance[288][288][288][288]in a two-dimensional system but did not believe that this was true. However, in 1980, Klaus Klitzing discovered that Hall conductance is exactly quantized in integers of [math]\frac{e^2}{h}[/math][289][289][289][289]through measurements of plateaus of the value of Hall resistance to an increasing magnetic field. The reason behind the exact quantization is from gauge invariance, as Laughlin found in 1981[290][290][290][290], but it was not clear as to why it was quantized.Laughlin came up with a fictitious magnetic field line that ran as a perpendicular loop to the conducting loop, which became the Laughlin quantum pump. The process would have a complete cycle every time the fictional flux increased by [math]\frac{h}{e}[/math], and once a cycle was over, the quantum system would return to the original state, allowing for gauge invariance. However, Hall conductance had been measured and averaged across many cycles, demonstrating that Laughlin’s assumption about the system being described by quantum mechanics was correct, but it meant that the original system did not have to be reproduced after each cycle. David Thouless extended Laughlin’s idea to a second fictitious magnetic field line, generating an adiabatic evolution that averaged over many cycles and showed that the average Hall conductance was quantized[291][291][291][291]. This allowed for the introduction of topology into understanding the quantization of the average Hall conductance, but it also broke Thouless’s argument. Experiments measured that the average Hall conductance was correlated with local curvature, not the average curvature.Later, Matthew Hastings and Xiao-Gang Wen began developing an idea in 2005 about gauge invariance being topological and unable to break down in the bosonic models[292][292][292][292][293][293][293][293]. Hastings continued on with the existence of a mobility gap in a many-body quantum system, which assumed that the motion of low energy fluctuations would be slow, and use that to create quasi-adiabatic continuation operators[294][294][294][294], which had been introduced in the last paper. Eventually, in 2015, Spyridon Michalakis solved the problem with Hastings, introducing two more fictitious magnetic field lines, transforming the adiabatic evolution under quasi-adiabatic continuation to a return to the original quantum state[295][295][295][295][296][296][296][296][297][297][297][297]. Michalakis realized that the use of adiabatic evolution to quantize Hall conductance was the reason behind the lack of progress. Adiabatic evolution requires the system to be at low energy throughout the cycle even when the energy of the system no longer corresponded with the lowest energy state.The integer quantum Hall effect does differ from the fractional quantum Hall effect, where there are plateaus at specific fractional values of [math]\frac{e^2}{h}[/math]. Under specific magnetic fields, the electron gas described by Fermi-Dirac statistics turns into a liquid that goes under quantum Hall transitions, forming plateaus at fractional values. The quasiparticles were first measured to have these fractional values in 1995 at Stony Brook[298][298][298][298], and then were measured to carry electric current in 1997[299][299][299][299][300][300][300][300].The fractional quantum Hall effect has different states of the same symmetry and may represent new states of matter existing in topological order. In fact, Hastings’s second paper briefly mentioned about the fractional quantum Hall effect, and he also added it to his paper co-authored by Michalakis. A better understanding of the fractional quantum Hall effect will help in understanding phase transitions in quantum systems.The quantum spin Hall effect is a proposed state that exists in two-dimensional semiconductors with quantized spin-Hall conductance. It was proposed by Charles Kane and Gene Mele in 2005[301][301][301][301][302][302][302][302], adapting Haldane’s earlier model of graphene exhibiting the integer quantum Hall effect[303][303][303][303]. In the proposed model, the spin-up electron has a chiral integer quantum Hall effect, while the spin-down electron has an achiral integer quantum Hall effect. Experiments cannot create this idealized system because the electrons are not coupled together, so better experiments will be necessary to create the quantum spin Hall effect.1981In 1981, Alan Guth theorized inflation. For the last two years, Guth had been working on the magnetic monopole problem and realized a false vacuum decay could explain the lack of monopoles in the observable universe[304][304][304][304]when most GUT theories predicted them. Guth proposed that the universe was cooling down in a false vacuum state at high energy densities, but the decay process only occurred by bubble nucleations caused by quantum tunneling. True vacuum states were being formed and spreading at the speed of light, but radiation could only occur when bubbles collided, and this would be rare if inflation lasted long enough to the point where any random region of spacetime would only have one bubble nucleating. Guth realized this issue in his model but could not find a way to allow for collisions between true vacuums states as inflation spread those states apart.However, Guth was not the only one at the time to theorize about inflation. The previous year, Alexei Starobinsky stated that quantum revisions to gravity would substitute the Big Bang singularity with an exponentially expanding de-Sitter space[305][305][305][305]. Later in 1980, Demosthenes Kazanas said that an exponential expansion could remove the particle horizon and resolve the horizon problem[306][306][306][306]of the cosmic microwave background. The CMB was isotropic in temperature on large scales, but this could not be possible because the largest distance within the background was too long for light to have traveled in the history of the universe, so the regions of spacetime furthest from each other could not have the same temperature unless an event occurred faster than light, the regions of spacetime were entangled, or spacetime itself had inflated. Following Guth’s paper, Katsuhiko Sato suggested that an exponential expansion would eliminate domain walls, which were theoretical dimensional singularities in spacetime that emit detectable gravitational waves[307][307][307][307]. Later, Sato and Martin Einhorn published a model similar to Guth’s inflation, stating it would solve the magnetic monopole problem but would require a fine-tuned cosmological constant that leads to observable density variations from collisions between true vacuum states[308][308][308][308].The bubble collision problem was first solved by Andrei Linde[309][309][309][309], and then independently by Andreas Albrecht and Paul Steinhardt[310][310][310][310]. There was no quantum tunneling out of the false vacuum under the slow-roll inflation, but there was a scalar field. The scalar field had potential energy, and when the field’s energy changed very little, inflation occurred, while when the field’s energy was changing rapidly, inflation ended, and the energy density of the inflation became fermionic matter. The new inflation model could explain the isotropic temperature of the cosmic microwave background and why observations showed it as homogenous, without the necessity of an event moving faster than light or an entangling of regions of spacetime.However, inflation did not create a perfectly symmetrical universe due to quantum fluctuations at the Planck scale before inflation, to create observable structures in the current universe. The fluctuations were first calculated by Viatcheslav Mukhanov and Gennady Chibisov in Starobinsky’s model[311][311][311][311][312][312][312][312]but applied to the new slow-roll inflation by Hawking[313][313][313][313], Starobinksy[314][314][314][314], Guth and So-Young Pi[315][315][315][315], and Steinhardt, James Bardeen, and Michael Turner[316][316][316][316]. Quantum fluctuations in the early universe were spread out by inflation, creating the anisotropy observed in the cosmic microwave background, and eventually, the galaxy superclusters in the observable universe.The theory of inflation predicts the collapse of the perturbations from quantum fluctuations during the period. The most thorough explanation is through a nearly-scale-invariant Gaussian random field that only has two free parameters, the amplitude of the spectrum, which measures the deviations that cause it to be nearly scale-invariant and the ratio of tensor to scalar, which the simplest models predict it to be 0.1[317][317][317][317][318][318][318][318]. Inflation also predicts that the perturbations should be in thermal equilibrium with each other in the cosmic microwave background, confirmed by measurements of the CMB[319][319][319][319][320][320][320][320]. The spectral index, the first parameter, is predicted to be between 0.92 and 0.98 by the simplest models[321][321][321][321]without the need for fine-tuning of parameters relating to energy[322][322][322][322][323][323][323][323]. Data from the Planck Telescope place the value at 0.968+-0.006 and a tensor to scalar ratio of less than 0.11[324][324][324][324][325][325][325][325]. These measurements seem to be confirmation for the inflation theory[326][326][326][326].A final test of inflation is of B-mode polarization in the cosmic microwave background, which would prove the existence of gravitational waves from the collapse of the perturbations predicted in inflation, and also to see if the energy levels for inflation given by the simplest models are correct. The first results in March 2014 found a tensor to scalar ratio between 0.15 and 0.27, but lower confidence was reported in June[327][327][327][327]and September[328][328][328][328][329][329][329][329]. In 2018, measurements calculated that the ratio was 0.06 or lower, but still consistent with the large number of models remaining[330][330][330][330]. However, most cosmologists still believe that inflation theory is correct and that better models will conform to future measurements.Unfortunately, widespread acceptance by cosmologists of the inflation theory does not mask the issues with some of the data in CMB measurements. Even though inflation does solve the magnetic monopole problem, the horizon problem, and the flatness problem, there is no other significant proof, such as the existence of the inflaton field, to absolutely confirm that inflation occurred in the early universe. Because of this, Steinhardt has become a vocal opponent of the theory he helped establish. Along with Anna Ijjas and Abraham Loeb, Steinhardt explained how measurements from the cosmic microwave background have already proven the simplest models incorrect and that more complex models would be less convincing as the reality that occurred in the early universe because of the requirement for fine-tuning of parameters relating to energy that the nearly-scale-invariant Gaussian random field did not make the free parameters[331][331][331][331][332][332][332][332]. Thus, Steinhardt proposed his simple models of ekpyrotic cyclic models and an anamorphic cosmology[333][333][333][333]. Under the ekpyrotic cyclic models, the horizon problem is solved in an epoch before the Big Bang in a previous universe, while the magnetic monopole is avoided by having the transition between Big Crunch to Big Bang periods have a lower temperature than predicted for the Grand Unified Epoch. The anamorphic model theorizes that a smoothing phase, solving the flatness problem, is when the Planck length is contracting, so that spacetime is expanding relative to Planck length, and a bounce occurs after the smoothing phase, further expanding spacetime and fixing values to the Compton wavelength and Planck mass[334][334][334][334]. Under these cosmological models, Steinhardt believes that they could better solve the problems Guth did in 1981 because of the simplicity of his models compared to the growing complexity of inflation models trying to conform with measurements of the cosmic microwave background[335][335][335][335].The trans-Planckian problem is also another issue with the theory of inflation. In black holes, the trans-Planckian problem deals with the fact that particles with a measurable finite wavelength and frequency will have an infinite frequency and zero wavelength at the event horizon. In inflation, the trans-Planckian problem does not deal with particles, but with regions of spacetime, since those regions of spacetime are predicted to be smaller than Planck length before inflation, causing the quantum fluctuations within those regions to be spread out by inflation into the structures we see today[336][336][336][336]. Those quantum fluctuations occurred in a distance smaller than Planck length, which makes them meaningless under known physics, but inflation allowed those fluctuations to become part of known physics by expanding their regions of spacetime in lengths larger than Planck length, making the fluctuations change spacetime to allow for matter-energy densities to vary between regions of spacetime, which became observable in measurements of the cosmic microwave background and structures in the current universe.1997In 1946, Hendrik Casimir and Dirk Polder proposed the existence of a force between two polarized atoms, and that this same force occurred between one of these atoms and a conducting plate[337][337][337][337], believing it may have some relation to London-van der Waals forces between atoms. They had noticed a slow development on the energy of interactions between neutral atoms, a retardation, which also occurred between a neutral atom and a conducting plate, so they decided to theorize on this effect via quantum electrodynamics[338][338][338][338][339][339][339][339]. Casimir discussed this with Bohr, who suggested that it may have to do with the zero-point energy of the quantum electrodynamic field, the lowest possible state of energy of the field, and Casimir incorporated this to his paper[340][340][340][340]on the interactions between two neutral conducting plates in a vacuum, where the plates still experience an attraction or repulsion because of virtual photons in the field in the region between the plates, creating a net force to act upon the plates because of the Casimir effect. However, the Casimir effect can be described without using the zero-point energy of the quantum electrodynamic field, by stating it as a relativistic and retarded van der Waals force between the conducting plates[341][341][341][341][342][342][342][342], which Casimir and Polder had originally use in their two papers. The Casimir force can only be measured successfully with small enough distances since the force rapidly decreases with distance, similar to the color force, but within these short distances, the force can be strong enough to produce a pressure of 1 atmosphere with the range of 10 nm between the two plates, although the value is dependent upon factors such as the geometry of the surfaces and the orientation of the plates.The Casimir effect was not fully confirmed until Steve Lamoreaux measured the Casimir force within a 5% error with theoretical predictions in 1997[343][343][343][343], although previous experiments had validated the theory with liquid helium films. A following paper measured with more accuracy, within the range of 0.1 to 0.9 μm[344][344][344][344][345][345][345][345], although both experiments used a parallel plate and a spherical plate so that it would be unnecessary to ensure the plates were parallel. However, in 2001, the Casimir effect was successfully measured between two parallel plates[346][346][346][346][347][347][347][347]by using microresonators.1998In 1998, it was discovered that the expansion of spacetime was accelerating rather than decelerating, as had been promoted for decades since Hubble’s observations in 1929. Cosmologists, from Eddington to Hawking, had believed that the expansion was decelerating, and possibly turn into a contraction that would force a Big Crunch to be the end of the universe. However, the last 6–7 billion years of the universe’s history had been dominated by dark energy accelerating the rate of expansion of spacetime, which went unnoticed by measurements since Hubble measured the redshift of the galaxies nearly 70 years ago, which caused cosmologists to believe that the universe was dominated by matter, which in turn, was believed to cause a gravitational collapse of spacetime into a Big Crunch[348][348][348][348][349][349][349][349][350][350][350][350][351][351][351][351][352][352][352][352], therefore, ending the universe. Unfortunately for cosmologists, the failure to measure accurately created serious misjudgment as to the state of the universe and where it was headed, so it came as a surprise when the expansion of spacetime was measured to be accelerating in 1998.The first team, led by Saul Perlmutter, demonstrated that the supernovae measured had a redshift larger than expected[353][353][353][353], so there had to be some other energy that existed causing the larger redshift, which in turn meant that the Hubble constant may be larger than expected, as some papers measured lower values[354][354][354][354][355][355][355][355][356][356][356][356][357][357][357][357][358][358][358][358][359][359][359][359]and others measured closer values[360][360][360][360][361][361][361][361][362][362][362][362][363][363][363][363][364][364][364][364][365][365][365][365]. Later that year, Adam Riess’s team confirmed the results of the first team, also using measurements of the redshifts of supernovae[366][366][366][366]. These results came as a shock to cosmologists, as they showed that the Hubble constant was increasing, which meant that there was some inherent energy within spacetime causing the expansion to accelerate.Until 2016, measurements of the Hubble constant were confirmed within their margins of error between those of Cepheid variables[367][367][367][367][368][368][368][368][369][369][369][369][370][370][370][370][371][371][371][371][372][372][372][372][373][373][373][373]and supernovae[374][374][374][374][375][375][375][375][376][376][376][376]to those of the cosmic microwave background[377][377][377][377][378][378][378][378][379][379][379][379][380][380][380][380][381][381][381][381], among other methods[382][382][382][382][383][383][383][383][384][384][384][384][385][385][385][385]. However, once the Planck 2013 results were published in 2014, there seemed to be an issue with calculations for the Hubble constant for that of the cosmic microwave background to the Cepheid variables and supernovae[386][386][386][386]. Calculations from the CMB measurements gave a value of 67.3 +- 1.2 km/s/Mpc, out of the range for the Cepheid variable and supernovae calculations of 74.3 +- 2.1 km/s/Mpc[387][387][387][387]. These different results for the Hubble constant became a “cosmological crisis” in the field[388][388][388][388]that had to be resolved. The difference did not improve in 2016, with Riess obtaining a result of 73.24 +- 1.74 km/s/Mpc[389][389][389][389]that confirmed earlier calculations with Cepheid variables and supernovae. Riess’s 2018 result further confirmed previous results, at a value of 73.48 +- 1.66 km/s/Mpc[390][390][390][390]. There was some help that the Cepheid variables and supernovae calculations would be reconciled with cosmic microwave background calculations later in the year when the Planck 2018 results were published, but those calculations came to a value of 67.4 +- 0.5 km/s/Mpc[391][391][391][391]that confirmed all previous CMB measurement values. Riess’s 2019 calculations shifted their result to 74.03 +- 1.42 km/s/Mpc[392][392][392][392], further away from CMB calculations, but the paper did point out the possibility of physics beyond the Standard Model affecting the ΛCDM model to be incorrect or incomplete and causing the difference in results between the Cepheid variables and supernovae calculations to the cosmic microwave background calculations.One possible method to decide which measurement was correct was by using red giants to calculate the Hubble constant. In the first paper after the Planck 2018 results, the value was calculated to be 69.8 +- 0.8 km/s/Mpc[393][393][393][393], and seemingly favored the calculations used in measuring the cosmic microwave background. However, Riess used the same method and calculated a value of 72.4 +- 2.0 km/s/Mpc[394][394][394][394], which seemed to favor the Cepheid variable and supernovae calculations. Because of this issue in calculations among red giants, we still have no idea whether the Cepheid variables and supernovae calculations or the cosmic microwave background calculations are genuinely correct. It is not out of possibility that physics beyond the Standard Model is interfering with measurements of the Hubble constant, but it seems unlikely at present. We know that the Hubble constant will increase in the future, but we must know what it is now to predict what it will be in the future when dark energy makes up a larger portion of the universe.In 2020, a new measurement down by the Atacama Cosmology Telescope of the cosmic microwave background calculated a Hubble constant of 67.6 +- 1.1 km/s/Mpc[395][395][395][395][396][396][396][396], which confirmed calculations done by the Planck team. Due to this result, it seems that measurements done for supernovae and Cepheid variables may have been miscalculated or had some type of error in measuring the distance or redshift. If that is the case, then it will become a severe but fixable problem in astronomy, as it may cause new measurements to be made for stars and galaxies to comply with the possible measurement error of supernovae and Cepheids. The error may be existent in only recent observations, which would not force astronomers to recalculate distances. It may also be an error in measuring the redshift, which would not likely cause a need to recalculate most distances.However, another measurement using kilonovae found two similar values using two different models. The first model had a value of 73.8 km/s/Mpc and the second model had a value of 71.2 km/s/Mpc[397][397][397][397], which both values confirm previous supernovae measurements of the Hubble constant, meaning that we still have a conflict between measurements of supernovae and measurements of the cosmic background radiation in the Hubble constant.Although there is a conflict over the Hubble constant, there is also an important we must understand about dark energy, specifically how it behaves. The current consensus in the community is that the equation of state, [math]w=\frac{p}{\rho}[/math], gives w a value of precisely -1[398][398][398][398]. However, there are some cosmologists who believe it is possible that w may have a value less than -1, which would create phantom dark energy, an idea most dismiss but don’t rule out[399][399][399][399]. Phantom dark energy was first proposed by Robert Caldwell in 2002, explaining the consequences of a value of w less than -1, the most important one being the Big Rip scenario[400][400][400][400][401][401][401][401]. Under phantom dark energy, the Big Rip would be the end of the universe, destroying all structure, down to the very quarks and electrons that compose all fermionic matter[402][402][402][402]. A recent book explains that if w is less than -1 but extremely close to -1, it extends the time until the Big Rip[403][403][403][403]. Currently, we do not know whether w is less than -1 or exactly equal to -1.2012In 2012, the Higgs boson was detected by the Large Hadron Collider. The Higgs boson, theorized by Peter Higgs and five others in 1964, was to solve the issue of spontaneous symmetry breaking in the electroweak theory, created by Glashow and Salam in 1959, while also avoiding the use of Goldstone bosons and keep the theory a gauge-invariant model. However, there had been no way to prove either theory correct until the detection of the W+/- and Z bosons in 1983, so after this point, it became necessary to detect the Higgs boson to ensure that the theory was correct in explaining spontaneous symmetry breaking, or otherwise, force Goldstone bosons to exist to explain an electroweak theory that was no longer covariant. How to detect the Higgs boson depended on its mass and Weinberg first theorized a lower bound of 3.72 GeV in 1976[404][404][404][404]putting experimental physics on the right track, knowing that the Higgs boson had a mass significantly larger than particles that had been previously detected, although not by much. Some had believed that it could be possible to detect the Higgs boson if it had a mass under 4 GeV[405][405][405][405], which would be detectable in particle collisions at the time, but as experiments continued and the W+/- and Z bosons were detected, the range of possible values of mass for the Higgs also increased, from above 63.9 GeV[406][406][406][406]to above 106.7 GeV[407][407][407][407]to above 114.4 GeV[408][408][408][408]. The consensus among physicists was that the Higgs boson could not have a mass higher than 200 GeV, and even though it was not directly possible to predict from the Standard Model what the mass of the Higgs boson could be, many physicists became sure after 2000, that the Higgs boson was close to being detected[409][409][409][409].The first significant search for the Higgs boson was at the Large Electron-Positron Collider in the 1990s, and by the time the LEP was closed in 2000, physicists were sure that the mass was above 114.4 GeV[410][410][410][410], but where remained debatable, since a range of 114.4 GeV to 200 GeV was still a considerable range to detect one boson and its interactions with other particles, including the fact that no previous detections had successfully found any events that may point a narrower range of mass for the Higgs boson. Fermilab continued the search in the Tevatron, which had famously detected the top quark in 1995[411][411][411][411][412][412][412][412][413][413][413][413][414][414][414][414], and although it did not succeed in finding the Higgs, it was able to exclude higher values of mass, from 147 GeV to 180 GeV. Some events were detected that indicated a possible mass between 115 GeV to 140 GeV[415][415][415][415], which proved inconclusive when the Tevatron shut down in 2011, but this helped the Large Hadron Collider focus detections in the range between 115 GeV and 140 GeV for possible events for interactions between particles of nonzero mass with the Higgs boson, but most notably with the W+/- and Z bosons.The ATLAS and CMS Collaborations had reported possible events in their data of proton-proton collisions in 2011, which directed a possible mass of the Higgs between 124 GeV and 126 GeV[416][416][416][416][417][417][417][417], confirmed by the last few experiments done in the Tevatron[418][418][418][418][419][419][419][419][420][420][420][420][421][421][421][421][422][422][422][422][423][423][423][423]before its closure. On 4 July 2012, the Higgs boson was confirmed to be detected in the Large Hadron Collider by both the ATLAS and CMS Collaborations[424][424][424][424][425][425][425][425], at a mass of 125.3/126 +- 0.4 GeV, finally confirming that the Higgs field was responsible for spontaneous symmetry breaking in electroweak theory and ensuring that gauge invariance remained. After the detection, physicists predicted how Standard Model particles would interact with the Higgs boson and how it would affect future experiments at high energies[426][426][426][426][427][427][427][427][428][428][428][428][429][429][429][429][430][430][430][430][431][431][431][431][432][432][432][432][433][433][433][433][434][434][434][434].The most prominent theory about the Higgs field is the false vacuum theory. The first theories about the false vacuum came in the late 1970s[435][435][435][435][436][436][436][436][437][437][437][437][438][438][438][438][439][439][439][439][440][440][440][440], one of which included the Higgs field[441][441][441][441], but mainly explained the process of how a collapse of the false vacuum state to the true vacuum state would occur once quantum tunneling had occurred within the energy density field. The collapse would occur in bubble nucleation and spread out across the universe at light-speed, creating a region of spacetime where the true vacuum existed and was stable, compared to outside the bubble, where the false vacuum existed, and spacetime was metastable. Bubble nucleation first appeared in Guth’s original formulation of inflation theory in 1981[442][442][442][442]but had been replaced by slow-roll inflation in 1982, causing the proposed scalar field of the inflaton to already be in a true vacuum state in the early universe, and only be in effect at the critical temperature when inflation was said to occur, but this immediately made questions about the false vacuum theory concerning the electroweak theory and the Higgs field.The stability of the energy density field of electroweak interactions was first theorized in 1979[443][443][443][443], but it focused mainly upon the mass of the Higgs, which was still unknown, and the mass of the top quark, which was also unknown. In connection with inflation theory[444][444][444][444], electroweak interactions could occur in a metastable state, since spontaneous symmetry breaking occurred at the same time as inflation[445][445][445][445]in the early universe. After the Higgs boson was detected, the theory that the electroweak force was metastable was considered again, but the mass of the Higgs was within range of the predicted mass necessary for vacuum stability[446][446][446][446]although the mass of the top quark was a factor necessary to be considered. Calculations between inflation theory and electroweak interactions continued because of the uncertainty about the mass of the top quark[447][447][447][447][448][448][448][448][449][449][449][449][450][450][450][450]could mean a stable vacuum or a metastable vacuum for electroweak interactions.As seen here, the stability of the vacuum depends on what the top quark’s mass is, which the Tevatron measured as about 173 GeV in 1995, but the uncertainty remains[451][451][451][451][452][452][452][452][453][453][453][453], although measurements in 2018 seem to confirm the masses of both the Higgs boson and the top quark, as well as that the electroweak force is in a false vacuum state[454][454][454][454]. However, physics beyond the Standard Model could change the above graph, possibly predicting that the electroweak force is in a true vacuum and stable, where quantum tunneling would not force the field into a different energy state since all other possible vacuum states are false vacuums and metastable[455][455][455][455][456][456][456][456].Even if the electroweak force was in a false vacuum state, the bubble could only expand at the speed of light and may never reach us because of the accelerating expansion of spacetime by dark energy. However, a false vacuum could collapse into a bubble relatively close to the earth since there is always a fluctuating possibility for the collapse to occur at any point in spacetime. In 2015, it was predicted that the rate for a vacuum decay was higher near black holes, creating a nucleation seed for a bubble[457][457][457][457], which meant that any collapse of a false vacuum could have occurred near primordial black holes, although a 2017 paper predicted that the bubble would collapse into the black hole rather than collapse out of it[458][458][458][458][459][459][459][459]. In 2020, it was found that, although small Schwarzschild black holes could increase the rate for a collapse, Kerr and Kerr-Newman black holes would stabilize the false vacuum and decrease the rate that would be expected for a flat curvature of spacetime[460][460][460][460].Under the false vacuum theory, the current false vacuum state is in de Sitter space, while the true vacuum state of the bubble is in Anti-de Sitter space. Of the three formulations of spacetime derived from special and general relativity, de Sitter space and Minkowski space are stable in the presence of matter-energy and gravity, so structures in their forms of spacetime can be formed without spacetime destabilizing and creating a black hole, when only gravity acts upon matter, or when only gravity is present. In de Sitter space, the cosmological constant is a positive value, and Ω is greater than 1, forming a spherical curvature for the universe, which should be what our universe’s type of space is. In Minkowski space, the cosmological constant is 0, and Ω is precisely equal to 1, forming a perfectly flat curvature of the universe, which is not our universe’s type of space even though measurements of spacetime seem to have Ω nearly equal to 1. In Anti-de Sitter space, the cosmological constant is a negative value, and Ω is less than 1, forming a hyperbolic curvature for the universe, which is also not our universe’s type of space, but the hyperbolic curvature is still possible since Ω may be larger than 1.In 1986, de Sitter space was proven to be stable in the presence of matter-energy and gravity[461][461][461][461], so a universe of de Sitter space should not have spacetime collapse into a black hole when matter-energy exists independent of forces beyond gravity or when gravity is being exerted without matter-energy or other forces being present. In 1993, Minkowski space was proven to be stable in the same conditions of matter-energy and gravity[462][462][462][462]affecting spacetime. However, Anti-de Sitter space was never proven stable or unstable, although many believed it was unstable. It was not until 2017 to 2020 that Anti-de Sitter space was proven unstable in the same conditions for matter-energy alone[463][463][463][463][464][464][464][464][465][465][465][465], where a universe of Anti-de Sitter space would have a spacetime that collapsed into a black hole if matter-energy existed and gravity was the only force that existed. There is no proof that Anti-de Sitter space is unstable in the sole presence of gravity, although it is highly likely that it is. It is this very reason about Anti-de Sitter space that tells us that, once vacuum decay occurs, life in the new universe is not possible because everything ultimately collapses into a black hole from the instability of spacetime in the new universe. This was explained in a famous paper by Sidney Coleman and Frank De Luccia in 1980[466][466][466][466][467][467][467][467], which was referenced in a recent book[468][468][468][468]. The existence of life is completely eliminated once vacuum decay occurs, because of the eventual gravitational effects that result in a black hole in the newly formed universe.Fortunately, the instability of spacetime in the new universe leaves the issue that the false vacuum theory predicts that the false vacuum state of the electroweak force has a spacetime more stable than the true vacuum state of the electroweak force, where black holes will form without any force beyond gravity. The universe would enter from a stable spacetime to an unstable spacetime that could potentially end the universe in a Big Crunch. Collapse from the false vacuum state would result in more than just the destruction of known physics within the universe; it would result destroy the universe itself. However, since the bubble of the true vacuum state can only expand at the speed of light, the destruction of spacetime could only occur within the bubble itself, so there would be no risk of spacetime breaking down outside the bubble, in the false vacuum state.2016In February 2016, LIGO detected gravitational waves for the first time, from a merging of a 36 solar mass and 29 solar mass black holes into a 62 solar mass black hole with 3 solar masses radiated through the detected gravitational waves[469][469][469][469], all from 1.3 billion light-years away. This detection was a necessary confirmation of general relativity since Einstein himself had doubted the existence of gravitational waves, but LIGO’s detection[470][470][470][470]opened the way for detections of other black hole mergers and collisions with and between neutron stars. In June, LIGO confirmed another pair of black holes colliding to form a 20.8 solar mass black hole[471][471][471][471]and 0.9 solar masses in gravitational waves. Four more detections of binary black hole mergers have been made since the second detection[472][472][472][472][473][473][473][473][474][474][474][474][475][475][475][475][476][476][476][476]in 2016, and there has also been at least one binary neutron star merger[477][477][477][477]in 2017, confirmed by electromagnetic observations. The second binary neutron star merger was not confirmed to be solely neutron stars, as the total mass of the system was 3.4 solar masses, more than any known binary neutron stars systems, which meant that one of the mergers could be a black hole[478][478][478][478], and the merging is likely the first detected between a black hole and a neutron star.However, the recent June 2020 confirmation has much greater significance because it deals with the mass gap between neutron stars and black holes, which has not been resolved in decades. The merger was made up of a 23 solar mass black hole and an object of 2.6 solar masses[479][479][479][479], which has yet to be confirmed as the most massive neutron star or the lightest black hole in the universe. The 2.6 solar mass object had been earlier confirmed as a neutron star due to its significantly smaller mass, but newer calculations put the object within the mass gap between neutron stars and black holes[480][480][480][480], and since neutron stars can only be as massive as 2.5 solar masses[481][481][481][481][482][482][482][482], while black holes should be as light as the unconfirmed 3.3 solar masses[483][483][483][483][484][484][484][484], we cannot confirm whether it is a neutron star or a black hole. Future developments on the merger may tell us which one it is or if this could be the first confirmed detection of the theorized quark star, a result of supernovae if gravity bypasses neutron degeneracy pressure but not the theorized quark degeneracy pressure[485][485][485][485][486][486][486][486][487][487][487][487]. The most recent calculations for the 2.6 solar mass object suggest that it cannot be a neutron star because it would be “inconsistent [with] either constraints obtained from energetic heavy-ion collisions or from the low deformability of medium-mass stars,” so the maximum mass for a neutron star cannot be significantly different from current observations and the 2.6 solar mass object must be the lightest black hole[488][488][488][488].2020In the last year, new experimental data has surfaced that may explain the matter-antimatter asymmetry of the early universe. After positrons were confirmed in 1932 by Anderson, a debate existed over the reason why antimatter was lacking throughout the universe and why the universe did not create an equilibrium between matter and antimatter previously, and the confirmation of the Big Bang by Penzias and Wilson in 1965 did nothing to relieve the situation, as it only proved further that asymmetry could not have formed in the Big Bang but by some other process yet to be known.The first explanation is the existence of CP violations by the decay of charm quarks more than the Standard Model predicts should occur[489][489][489][489]between charm quarks and charm antiquarks. CP violations are predicted by the Standard Model, but only up to a certain extent for each particle that can decay, so any significantly larger number than usual could mean that the matter-antimatter asymmetry could be explained for baryons. Current experiments suggest that events of CP violation are occurring more often than prediction, where the CP violation events in the decay of the charm quark are forming more matter particles than would be in the decay of the charm antiquark, which would help solve the baryon asymmetry in the early universe when charm quarks and charm antiquarks would have existed. However, since the five σ threshold necessary to constitute a discovery has yet to be passed, more experiments will be necessary to ensure that baryon asymmetry can be explained by the decay of charm quarks[490][490][490][490].The second is the interactions between the Higgs boson and fermions. Interactions between the Higgs boson and quarks may cause CP violations, which would mean a difference in the decays of quarks and antiquarks based on how the Higgs boson interacts with them. Current experiments involving interactions between the top quark and the Higgs boson have seen no CP violations to date[491][491][491][491], but interactions between other quarks and the Higgs may explain the baryon asymmetry.The third is CP violation in neutrino oscillations and antineutrino oscillations, which could explain leptonic asymmetry. Both neutrinos and antineutrinos oscillate between flavors, from electron, to muon, to tau, in any order. The asymmetry observed in their oscillations may explain the matter-antimatter asymmetry among leptons during the Lepton Epoch[492][492][492][492][493][493][493][493]along with the baryon asymmetry from other causes. This may be complicated further by the existence of the sterile neutrino, a candidate for dark matter, which will be explained in a following section.There have also been five less likely explanations for the existence of the matter-antimatter asymmetry, an inflation-driven change in the Higgs field that caused leptogenesis[494][494][494][494], the existence of more scalar fields that interacted with fermions[495][495][495][495][496][496][496][496], inflation causing baryon asymmetry[497][497][497][497][498][498][498][498], primordial black holes through their formation[499][499][499][499]or evaporation[500][500][500][500]via Hawking radiation, and “hidden” dark matter as antimatter[501][501][501][501][502][502][502][502]. However, the most promising ideas to explain matter-antimatter asymmetry is through CP violations of charm quark decays, interactions between quarks and the Higgs boson, and asymmetrical neutrino and antineutrino oscillations.2020Recently, there was a reported dark matter detection at the XENON1T experiment, where there was an excess of events within the liquid xenon, that could either be caused by contamination of tritium, dark matter WIMPS, solar axions, or the existence of magnetic moments in neutrinos[503][503][503][503][504][504][504][504][505][505][505][505][506][506][506][506][507][507][507][507]. Researches at XENON1T believed that the data most matched with the existence of solar axions[508][508][508][508]and point towards another axion as dark matter. However, both solar axions and neutrinos with magnetic moments have an issue with known astronomical observations of the lives of white dwarves and giants, since both particles are theorized to take energy away from a star when they leave the star, which would mean white dwarves and giants would have shorter lifetimes than astronomers suggest. That does not mean they cannot exist since new physics will be at play in both solar axions and neutrinos, as well as help us understand dark matter better. Current data suggest that solar axions are the best explanation for the observation of excess events, although it is still at the 3.5 σ level, not past the five σ threshold necessary for discovery[509][509][509][509]. Other researchers have also suggested how axion dynamics describes dark matter and its effects[510][510][510][510][511][511][511][511].However, the idea of axions came from a different origin, the strong CP problem of quantum chromodynamics, where CP violations are predicted in theory but not observed in experiments[512][512][512][512]. Under the Lagrangian form, there are natural terms that can cause CP violations, especially the nonzero values of θ and θ’, which means if one of the quarks was massless, such as the up quark, the lightest of all six quarks, θ would become unobservable or have a value of 0, and then the absence of CP violations would be in quantum chromodynamics. However, experiments have shown that all quarks have a measurable mass and that θ can be observed, while never actually measured, so CP violations must be predicted by quantum chromodynamics even though CP symmetry is also observed.[math]L = -\frac{F_{μv}F^{μv}}{4} - \frac{n_{f}g^{2}θ}{32π^2}F_{μv}\overline{F}^{μv} [/math][math][/math][math]+ \overline{ψ}(iγ^{μ}D_{μ} - me^{iθ`γ_5})ψ[/math]In 1977, Peccei-Quinn theory proposed a global symmetry of U(1) that would be spontaneously broken, where θ is predicted to have a small value nearly equal to 0[513][513][513][513]and the axion is the particle that results from the spontaneous symmetry breaking of the theory, as Wilczek and Weinberg theorized[514][514][514][514]. The axion would be a Goldstone boson, which had been theorized before the Higgs, to explain spontaneous symmetry breaking of the electroweak theory and force it to be no longer gauge invariant. However, vacuum effects under quantum chromodynamics are not truly symmetrical, forcing the axion to have mass, which means that it is not a true Goldstone boson, but a pseudo-Goldstone boson, ruling out Wilczek and Weinberg’s original axion. The invisible axion finally brought dark matter into the mix, where the axion could both explain dark matter and solve the strong CP problem simultaneously. The invisible axions would interact very weakly in experiments as a WIMP, explaining why there was no detection in experiments trying to detect dark matter or CP violations[515][515][515][515][516][516][516][516][517][517][517][517]. However, because of their light mass, there had to be a specific range of masses, or else there would have been enough created in the early universe to be detected, so the critical mass is [math]10^{-11}[/math] times the electron’s mass[518][518][518][518][519][519][519][519][520][520][520][520], which makes it a suitable candidate for dark matter. The most recent calculations put the mass of the axion between 0.05 and 1.5 MeV[521][521][521][521]. The most current research is using trying to detect interactions between axions and a magnetic field that create an oscillating magnetic field and using magnets to find these oscillating fields[522][522][522][522][523][523][523][523].2020As previously mentioned, neutrinos may have a sterile flavor, a fourth flavor, that exists beyond the current Standard Model. The sterile neutrino may link us to dark matter and dark energy, since they interact only through gravity, rather than through strong or weak interactions that the other flavors do. In the 1990s, the Liquid Scintillator Neutrino Detector at Los Alamos observed more neutrinos than expected from a beam of muon neutrinos, since the distance was not long enough for most muon neutrinos to change flavor into electron neutrinos unless some oscillated into a fourth sterile neutrino in the process[524][524][524][524]. The results of the experiment showed that some fourth neutrino must exist to allow for this oscillation between muon and electron flavors to occur in a short time[525][525][525][525][526][526][526][526][527][527][527][527][528][528][528][528]. Fermilab’s 2018 results confirmed the excess of electron neutrinos in their 15-year experiment at the six σ level[529][529][529][529], which meant that some event was occurring to cause the oscillations and oscillation to a fourth flavor seemed the most likely cause since neutrinos rarely ever interact with other fermions. The Coherent CAPTAIN-Mills Experiment at Los Alamos will try to provide more evidence for sterile neutrinos exist, along with other experiments[530][530][530][530]trying to find evidence for the fourth neutrino. In theory, a neutrino that oscillates into the fourth flavor would seemingly disappear to an observer moving at the same speed, and then the neutrino would reappear in one of the three flavors, although it may be possible that the sterile neutrino could decay into other particles, ending the oscillation cycle. If the sterile neutrino can decay into other particles, this could help solve the matter-antimatter asymmetry, both the baryon and leptonic forms of the asymmetry[531][531][531][531]. Including CP violations in the oscillations between neutrinos and antineutrinos, matter-antimatter asymmetry could be well explained through how the physics of the neutrino, on and beyond the Standard Model. However, the most recent experiment on sterile neutrinos placed significant constraints on the angle θ for finding sterile neutrinos[532][532][532][532], causing some to doubt the existence of sterile neutrinos and the overall idea of a fourth flavor of neutrinos.2020Currently, it is now suggested that the black hole information paradox maybe be solved[533][533][533][533]. The solution comes from the Page curve that Page theorized in place of Hawking's entropy calculations[534][534][534][534]due to a split in their views on how the no-hair theorem worked in the context of Hawking radiation[535][535][535][535][536][536][536][536]. Page realized the important point of quantum entanglement had been neglected when considering what happens as the black hole evaporates towards its death. While this issue was eventually solved by the AMPS firewall in 2012 while violating the equivalence principle, Page had come up with a different way of removing the entanglement entropy of the black hole system by reasoning that it increases until a particular time called Page time, where the black hole is still massive but it has reached about halfway through its life. There was no process that could justify the entanglement entropy decreasing at Page time, but Page’s solution allowed for information to leave the black hole via Hawking radiation.Now, the issue came to mathematically proving whether the Page curve was correct and if it was possible to do away with the AMPS firewall paradox altogether. This started in 2018 with Ahmed Almheiri using a quantum error correction of AdS/CFT[537][537][537][537], then continued with a string theory concept[538][538][538][538]developed by Juan Maldacena in 2019. At the same time, Geoffrey Penington used a different method with AdS/CFT[539][539][539][539]but also showed how the entropy was always less than the one predicted by Bekenstein and Hawking, as well as avoiding the AMPS firewall paradox. Because black holes do not evaporate in AdS/CFT, Almheiri and others had to apply a method[540][540][540][540]to remove the radiation from space in order to allow for evaporation. Next, they theorized a method to track the entanglement entropy using a paper co-author Netta Engelhardt wrote on calculating the entropy for a quantum extremal surface[541][541][541][541].The surface carves the bulk into two parts, one that matches with the event horizon, the other that matches with the quantum extremal surface, while the area of the surface is proportional to the entanglement entropy in between, so the entanglement entropy rises until Page time, when the quantum extremal surface appears under the event horizon and eventually causes the drop in entropy. The group was able to track the entanglement entropy using Engelhardt’s method to what Page predicted, confirming that information was released by Hawking radiation, even though the information is encrypted due to quantum entanglement and it appears that the radiation released means nothing. They were able to express quantum entanglement geometrically in AdS/CFT space[542][542][542][542], which then could be extended to the real universe, and was confirmed for Schwarzschild black holes within a flat geometry in April 2020[543][543][543][543].Next, the group found that both the black hole and the Hawking radiation follow the same Page curve[544][544][544][544][545][545][545][545], which implies that information is transferred from the black hole to the radiation, but it is not clear how the information is transferred. However, the calculations also say that particles inside the black hole no longer contribute to the entanglement entropy, which explains why that decreases, but the particles are somehow part of the radiation. The authors referred to this group of particles as the “island” inside the black hole, but not of the black hole, and therefore not part of the entanglement entropy. This seemingly paradoxical idea has not been solved.Then, there was the issue of generalizing the Page curve to all black holes, which was solved by using Feynman’s path integral[546][546][546][546][547][547][547][547], but this methodology still failed to explain how the information was transferred. Wormholes could be a possible solution due to quantum entanglement as Maldacena and Susskind theorized in 2013[548][548][548][548]and allow for information to be transferred from the black hole to the radiation, but others argue that wormholes should not be considered in order for the path integral to make sense[549][549][549][549]. Many, including the authors, believe that the black hole information paradox has not been solved completely due to the issues of how the information is transferred and what it means for particles to be in the “island” instead of the black hole. A successful theory of quantum gravity was considered the best solution to the information paradox, and the authors still consider quantum gravity the solution unless there is a way to understand and solve the paradoxes brought up by the AdS/CFT method. For now, all we can say is that we have no idea how information is transferred from the black hole to the radiation, or what it means for the “island” to be inside the black hole but not of the black hole.2021The no-hair theorem may be in trouble, now that detecting hair could be possible, through observing theorized gravitational instabilities at the event horizon, specifically those of near-extremal black holes[550][550][550][550]. A paper in 2013[551][551][551][551]suggested that some black holes may have gravitational instabilities at the event horizon may cause regions of the horizon to have a larger gravitational effect than other regions, therefore allowing black holes to be distinguished between each other[552][552][552][552]. However, the derived equations only worked for extremal black holes, where values of mass, charge, or spin are at a maximum, and such black holes are not predicted to exist in the universe. On the other hand, near-extremal black holes should exist, in which it would be possible to detect violations to the no-hair theorem, and, unsurprisingly, hair could be measured through observations of gravitational waves. The authors of the January paper suggested that disturbances in the formation or infalling material would cause instabilities on or around the event horizon of a near-extremal black hole, therefore creating gravitational waves different than would be observed from ordinary black holes[553][553][553][553]. However, there are the two issues of whether near-extremal black holes actually exist, since simulations have only reached 30% from being extremal, and if gravitational wave detectors can find the differences caused by hair, since the hair last less than a second. The hope is that the detectors are sensitive enough to find those differences and whether we can interpret them correctly, which would prove the existence of near-extremal black holes, disprove the no-hair theorem, and further solve the black hole information paradox.Future Developments in Quantum Physics and CosmologyBased on current events in the field, we will soon discover what dark matter is and what will explain matter-antimatter asymmetry. We may also find the sterile fourth flavor of neutrinos, pushing us out of the Standard Model[554][554][554][554][555][555][555][555], and the axions of dark matter. Eventually, we will have to find a way to combine quantum mechanics with general relativity to form a theory of quantum gravity[556][556][556][556][557][557][557][557]that can explain gravity’s inherent weakness in the quantum world and possibly how black holes work[558][558][558][558]and quite possibly the singularities within black holes and the Big Bang singularity. This may be through string theory[559][559][559][559][560][560][560][560][561][561][561][561][562][562][562][562][563][563][563][563][564][564][564][564][565][565][565][565][566][566][566][566][567][567][567][567]or loop quantum gravity[568][568][568][568][569][569][569][569][570][570][570][570][571][571][571][571][572][572][572][572][573][573][573][573][574][574][574][574][575][575][575][575][576][576][576][576][577][577][577][577][578][578][578][578][579][579][579][579][580][580][580][580][581][581][581][581][582][582][582][582][583][583][583][583], or the many theories still out there or have yet to exist. (I do not like either string theory or LQG myself.) We will theorize a successful GUT[584][584][584][584][585][585][585][585][586][586][586][586]to combine quantum chromodynamics with the electroweak force and combine this theory with quantum gravity to give us TOE[587][587][587][587], helping us explain what occurs in the singularity of a black hole and the Big Bang itself. It will also combine dark energy and axionic dark matter into the theory, as well as the Higgs field, giving us a clear description of the laws of physics in our universe, as well as help us predict events with better accuracy even with the uncertainty that always remains.Heat Death of the UniverseBillions of years from now, when the last black hole has finally evaporated from Hawking radiation, the universe will enter a heat death. The entire universe will be at thermal equilibrium everywhere, and no process can use energy at this stage[588][588][588][588]since equilibrium exists. As spacetime expands from dark energy, the total matter-energy density decreases with time, to the point where the density is equal everywhere in the universe, creating an equilibrium. The entropy of the universe will have increased to a maximum at this stage, after consistently increasing since the Big Bang, as must be necessary to follow the Second Law of thermodynamics. This would prove that the universe is an isolated system where entropy must always increase overall[589][589][589][589][590][590][590][590][591][591][591][591][592][592][592][592][593][593][593][593][594][594][594][594][595][595][595][595], even when some events decrease the entropy on their own. The universe will stay this way infinitely, until some other process independent of the energy density, occurs, possibly changing our universe or even creating a new universe.Sources:Evolution of the Universe through the Planck epochCosmic Evolution - Epoch 1 - Particle EvolutionGrand Unified Models and CosmologyAn Introduction to Spontaneous Symmetry BreakingThe Search for the Quark-Gluon PlasmaThe Quark-Gluon Plasma in EquilibriumPhysical processes in the universe at the epoch of the quark-hadron transitionQuark-hadron phase transition in the early Universe: Isothermal baryon-number fluctuations and primordial nucleosynthesisThe matter-antimatter asymmetry problemNeutrino decoupling in the early UniverseNeutrino masses and the cosmic-neutrino backgroundPrecision Detection of the Cosmic Neutrino BackgroundConstraints on the cosmic neutrino backgroundA First Detection of the Acoustic Oscillation Phase Shift Expected from the Cosmic Neutrino BackgroundBaryogenesis via leptogenesisResonant LeptogenesisLeptogenesis as the Origin of MatterLeptogenesisBig-Bang Nucleosynthesis RevisitedBig-Bang Nucleosynthesis RevisitedPrimordial Nucleosynthesis ReduxPrimordial Nucleosynthesis: Theory and ObservationsPlanck and the cosmic microwave backgroundThe Dark Ages of the UniverseFormation of the First Stars and GalaxiesType Ia Supernova Discoveries at Z > 1 from the Hubble Space Telescope: Evidence for Past Deceleration and Constraints on Dark Energy EvolutionDark Energy | Definition of Dark Energy by Oxford Dictionary on Lexico.com also meaning of Dark EnergyThe Mathematical Principles of Natural PhilosophyII. The Bakerian Lecture. On the theory of light and coloursIllustrations of the Dynamical Theory of Gases. - Part I. On the Motions and Collisions of Perfectly Elastic Spheres.Illustrations of the Dynamical Theory of Gases. - Part II. On the Process of Diffusion of two or more kinds of moving particles among one another.Weitere Studien über das Wärmegleichgewicht unter GasmolekülenÜber die Beziehung zwischen dem zweiten Hauptsatze des mechanischen Wärmetheorie und der Wahrscheinlichkeitsrechnung, respective den Sätzen über das Wärmegleichgewicht.Translation of Ludwig Boltzmann’s Paper “On the Relationship between the Second Fundamental Theorem of the Mechanical Theory of Heat and Probability Calculations Regarding the Conditions for Thermal Equilibrium”A Dynamical Theory of the Electromagnetic Field. [Abstract]A Dynamical Theory of the Electromagnetic FieldOn a New Action of the Magnet on Electric CurrentsOn a New Action of the Magnet on Electric CurrentsXL. Cathode Rays.Influence of Motion of the Medium on the Velocity of LightOn the Relative Motion of the Earth and the Luminiferous EtherLorentz Invariance Still StandsConstraints on Lorentz Invariance Violation from HAWC Observations of Gamma Rays above 100 TeVConstraints on Lorentz Invariance Violation from HAWC Observations of Gamma Rays above 100 TeV (pdf)Physicists Have Tested The Speed of Light at The Highest Energies YetOn the Theory of the Energy Distribution Law of the Normal SpectrumOn a Heuristic Point of View about the Creation and Conversion of Light (Original Paper)On a Heuristic Point of View about the Creation and Conversion of Light (English Translation)On the Electrodynamics of Moving BodiesDoes the Inertia of a Body Depend Upon Its Energy Content?The Foundation of the General Theory of RelativityOn Gravitational WavesOn a Stationary System With Spherical Symmetry Consisting of Many Gravitating MassesA Determination of the Deflection of Light by the Sun's Gravitational Field, from Observations Made at the Total Eclipse of May 29, 1919The Total Eclipse of 1919 May 29 and the Influence of Gravitation on LightRecherches sur la théorie des quanta (Researches on the quantum theory)The wave nature of the electron (de Broglie’s 1929 Nobel Lecture)Diffraction of Cathode Rays by a Thin FilmReflection of Electrons by a Crystal of NickelElektroneninterferenzen an mehreren künstlich hergestellten FeinspaltenThe Young-Feynman two-slits experiment with single electrons: Build-up of the interference pattern and arrival-time distribution using a fast-readout pixel detectorPlanck’s law and the hypothesis of light quantaPlancks Gesetz und LichtquantenhypothesePlanck's Law and the Light Quantum HypothesisOn the Connexion between the Completion of Electron Groups in an Atom with the Complex Structure of SpectraThe Connection Between Spin and StatisticsQuantum-Theoretical Re-interpretation of Kinematic and Mechanical RelationsOn Quantum MechanicsÜber das Wasserstoffspektrum vom Standpunkt der neuen Quantenmechanik (About the hydrogen spectrum from the point of view of the new quantum mechanics)Quantisierung als Eigenwertproblem (first paper)Collected Papers on Wave MechanicsAn Undulatory Theory of the Mechanics of Atoms and MoleculesZur Quantenmechanik der Stoßvorgänge (Quantum mechanics of collision processes)Quantum mechanics of collision processesOn the Quantization of the Monoatomic Ideal GasOn the theory of quantum mechanicsÜber den anschaulichen Inhalt der quantentheoretischen Kinematik und MechanikZur Quantenmechanik einfacher BewegungstypenThe Quantum Theory of the Emission and Absorption of RadiationThe quantum theory of the emission and absorption of radiationOn the quantum electrodynamics of charge-free fieldsZur Quantenelektrodynamik ladungsfreier FelderQuantum Theory of RadiationQuantum Theory of RadiationConsequences of Dirac’s Theory of the PositronFolgerungen aus der Diracschen Theorie des PositronsUn Univers homogène de masse constante et de rayon croissant rendant compte de la vitesse radiale des nébuleuses extra-galactiques (A Homogeneous Universe of Constant Mass and Increasing Radius accounting for the Radial Velocity of Extra-galactic Nebulæ)Homogeneous Universe of Constant Mass and Increasing Radius accounting for the Radial Velocity of Extra-galactic NebulæThe Beginning of the World from the Point of View of Quantum TheoryThe Quantum Theory of the ElectronThe Quantum Theory of the Electron. Part IIThe Positive ElectronA Relation Between Distance and Radial Velocity Among Extra-Galactic NebulaeThe Expanding Universe (Clark)The Expanding Universe (Eddington)The Large Numbers hypothesis and the Einstein theory of gravitationThe Future of the UniverseSteps Toward the Hubble Constant. V. The Hubble Constant from Nearby Galaxies and the Regularity of the Local Velocity FieldSteps Toward the Hubble Constant. VI. The Hubble Constant Determined from Redshifts and Magnitudes of Remote Sc I Galaxies: The Value of qSteps Toward the Hubble Constant. VIII. The Global ValueOrigin of the Hubble constant controversySurface Brightness Fluctuations: A Bridge from M31 to the Hubble ConstantThe Hubble constant and Virgo cluster distance from observations of Cepheid variablesUsing SN Ia Light Curve Shapes to Measure The Hubble ConstantDetermination of the Hubble constant from observations of Cepheid variables in the galaxy M96Measuring the Hubble Constant with the Hubble Space TelescopeQuantised Singularities in the Electromagnetic FieldQuantised singularities in the electromagnetic fieldEvidence for Detection of a Moving Magnetic MonopoleFirst Results from a Superconductive Detector for Moving Magnetic MonopolesTheoretical and Experimental Status of Magnetic MonopolesCosmological Production of Superheavy Magnetic MonopolesPhase Transitions and Magnetic Monopole Production in the Very Early UniverseSupercooled phase transitions in the very early universeMagnetic MonopolesDie Rotverschiebung von extragalaktischen NebelnThe Redshift of Extragalactic NebulaeOn the Masses of Nebulae and of Clusters of NebulaeCan Quantum-Mechanical Description of Physical Reality Be Considered Complete? (Einstein, Podolsky, Rosen)Can Quantum-Mechanical Description of Physical Reality be Considered Complete? (Bohr)Physik und realitätPhysics and realityA Suggested Interpretation of the Quantum Theory in Terms of "Hidden" Variables. IA Suggested Interpretation of the Quantum Theory in Terms of "Hidden" Variables. IIDie gegenwärtige Situation in der Quantenmechanik.The Present Situation in Quantum MechanicsThe Origin of Chemical ElementsNote on the Theory of the Interaction of Field and MatterNote on the Radiation Field of the ElectronOn the Self-Energy and the Electromagnetic Field of the ElectronFine Structure of the Hydrogen Atom by a Microwave MethodOn the Intrinsic Moment of the ElectronThe Electromagnetic Shift of Energy LevelsOn a Relativistically Invariant Formulation of the Quantum Theory of Wave Fields.On Quantum-Electrodynamics and the Magnetic Moment of the ElectronQuantum Electrodynamics. I. A Covariant FormulationQuantum Electrodynamics. II. Vacuum Polarization and Self-EnergyQuantum Electrodynamics. III. The Electromagnetic Properties of the Electron---Radiative Corrections to ScatteringThe Radiation Theories of Tomonaga, Schwinger, and FeynmanThe S Matrix in Quantum ElectrodynamicsThe Theory of PositronsSpace-Time Approach to Quantum ElectrodynamicsMathematical Formulation of the Quantum Theory of Electromagnetic InteractionÜber den Bau der Atomkerne. IOn the Consequences of the Symmetry of the Nuclear Hamiltonian on the Spectroscopy of NucleiCharge Independence for V -particlesCharge Independence Theory of V ParticlesThe Interpretation of the New Particles as Displaced Charge Multiplets.Synthesis of the Elements in StarsOn Nuclear Reactions Occurring in Very Hot Stars. I. the Synthesis of Elements from Carbon to NickelThe Synthesis of the Elements from HydrogenEnergy Production in StarsStellar Evolution, Nuclear Astrophysics, and NucleogenesisNeutron capture chains in heavy element synthesisNucleosynthesis of Heavy Elements by Neutron Capture.Nucleosynthesis During Silicon BurningNuclear Quasi-Equilibrium during Silicon BurningQuestion of Parity Conservation in Weak InteractionsExperimental Test of Parity Conservation in Beta DecayThe Renormalizability of Vector Meson InteractionsWeak and Electromagnetic Interactions.The Eightfold Way: A Theory of Strong Interaction SymmetryA Schematic Model of Baryons and MesonsAn SU(3) Model for Strong Interaction Symmetry and Its BreakingAn SU(3) Model for Strong Interaction Symmetry and Its Breaking IIMagnetic moments of baryons in a quark modelA contribution to the history of quarks: Boris Struminsky's 1965 JINR publicationSpin and Unitary-Spin Independence in a Paraquark Model of Baryons and MesonsThree-Triplet Model with Double SU(3) SymmetryPlasmons, Gauge Invariance, and MassBroken Symmetry and the Mass of Gauge Vector MesonsBroken Symmetries and the Masses of Gauge BosonsGlobal Conservation Laws and Massless ParticlesField theories with "Superconductor" solutionsOn the Einstein Podolsky Rosen ParadoxThe Free-Will Postulate in Quantum MechanicsEntangled quantum states in a local deterministic theorySpeakable and Unspeakable in Quantum MechanicsExperimental Test of Local Hidden-Variable TheoriesExperimental Tests of Realistic Local Theories via Bell's TheoremExperimental Test of Bell's Inequalities Using Time-Varying AnalyzersExperimental demonstration of quantum correlations over more than 10 kmViolation of Bell Inequalities by Photons More Than 10 km ApartViolation of Bell's Inequality under Strict Einstein Locality ConditionsExperimental test of quantum nonlocality in three-photon Greenberger–Horne–Zeilinger entanglementExperimental violation of a Bell's inequality with efficient detectionExperimental violation of a Bell's inequality with efficient detectionAn experimental test of non-local realismErratum: An experimental test of non-local realismAn experimental test of non-local realismSpacelike Separation in a Bell Test Assuming Gravitationally Induced CollapsesSpace-like Separation in a Bell Test assuming Gravitationally Induced CollapsesViolation of Bell's inequality in Josephson phase qubitsBell violation using entangled photons without the fair-sampling assumptionBell violation with entangled photons, free of the fair-sampling assumptionDetection-Loophole-Free Test of Quantum Nonlocality, and ApplicationsDetection-Loophole-Free Test of Quantum Nonlocality, and ApplicationsBell-inequality violation with entangled photons, free of the coincidence-time loopholeBell violation with entangled photons, free of the coincidence-time loopholeLoophole-free Bell inequality violation using electron spins separated by 1.3 kilometresExperimental loophole-free violation of a Bell inequality using entangled electron spins separated by 1.3 kmSignificant-Loophole-Free Test of Bell’s Theorem with Entangled PhotonsStrong Loophole-Free Test of Local RealismBell Correlations in a Bose-Einstein CondensateCosmic Bell Test: Measurement Settings from Milky Way StarsEvent-Ready Bell Test Using Entangled Atoms Simultaneously Closing Detection and Locality LoopholesChallenging local realism with human choicesPublisher Correction: Challenging local realism with human choicesChallenging local realism with human choicesCosmic Bell Test Using Random Measurement Settings from High-Redshift QuasarsA Measurement of Excess Antenna Temperature at 4080 Mc/sCosmic Black-Body RadiationA New Model for the Expanding UniverseA Quasi-Steady State Cosmological Model with Creation of MatterAstrophysical deductions from the quasi-steady-state cosmologyErratum: Astrophysical deductions from the quasi-steady-state cosmologyFurther astrophysical quantities expected in a quasi-steady state UniverseThe basic theory underlying the quasi-steady-state cosmologyInterpretations of the Accelerating UniverseComments on the quasi-steady-state cosmologyNote on a Comment by Edward L. WrightErrors in the Steady State and Quasi-SS ModelsPerturbations of a Cosmological Model and Angular Variations of the Microwave BackgroundLarge-scale Density Inhomogeneities in the UniverseSmall-Scale Fluctuations of Relic RadiationThe Sunyaev–Zeldovich effect towards three clusters of galaxiesCosmic Microwave Background Dipole Spectrum Measured by the COBE FIRAS InstrumentDMR Data ProductsDetection of polarization in the cosmic microwave background using DASIDetection of Polarization in the Cosmic Microwave Background using DASIPolarization Observations with the Cosmic Background ImagerPolarization Observations with the Cosmic Background ImagerA Model of LeptonsNeutrino PhysicsExperimental study of structure functions and sum rules in charge-changing interactions of neutrinos and antineutrinos on nucleonsTransverse Mass and Width of the W BosonResults from the UA1 and UA2 Experiments.The Discovery of the W and Z ParticlesEvent Horizons in Static Vacuum Space-TimesEvent horizons in static electrovac space-timesAxisymmetric Black Hole Has Only Two Degrees of FreedomGravitationGeonsRotation of the Andromeda Nebula from a Spectroscopic Survey of Emission RegionsExtended HI Rotation Curve and Mass Distribution of M31A Comparison of Dynamical Models of the Andromeda Nebula and the GalaxyComparison of Rotation Curves of Different Galaxy TypesDynamics of Early Type Galaxies. I. The Rotation Curve of the Elliptical Galaxy NGC 4697Rotation curve of the edge-on spiral galaxy NGC 5907: disc and halo massesThe Universal Galaxy Rotation CurveSignatures of Galaxy-Cluster Interactions: Spiral Galaxy Rotation Curve Asymmetry, Shape, and ExtentThe Time Delays of Gravitational Lens HE 0435-1223: An Early Type Galaxy with a Rising Rotation CurveBullet Cluster: A Challenge to ΛCDM CosmologyThe rise and fall of a challenger: the Bullet Cluster in Λ cold dark matter simulationsThe Behavior of Hadron Collisions at Extreme EnergiesHigh-Energy Inelastic e − p Scattering at 6° and 10°Observed Behavior of Highly Inelastic Electron-Proton ScatteringConservation of Isotopic Spin and Isotopic Gauge InvarianceAdvantages of the Color Octet Gluon PictureUltraviolet Behavior of Non-Abelian Gauge TheoriesReliable Perturbative Results for Strong Interactions?Confinement of quarksHigh-Precision Lattice QCD Confronts ExperimentHigh-Precision Lattice QCD Confronts ExperimentQuantum Yang-Mills TheoryBlack hole explosions?Particle creation by black holesParticle emission rates from a black hole: Massless particles from an uncharged, nonrotating holeDo Evaporating Black Holes Form Photospheres?Universal upper bound on the entropy-to-energy ratio for bounded systemsDo Black Holes Destroy Information?Soft Hair on Black HolesSoft Hair on Black HolesBlack Holes Have Soft Quantum HairOn the quantum structure of a black holeBlack-hole evaporation and ultrashort distancesHawking radiation without trans-Planckian frequenciesHawking Radiation Without Transplanckian FrequenciesInformation in black hole radiationInformation in Black Hole RadiationAverage entropy of a subsystemAverage Entropy of a SubsystemBlack holes: complementarity or firewalls?Black Holes: Complementarity or Firewalls?An Apologia for FirewallsGauge-Gravity Duality and the Black Hole InteriorAstrophysics: Fire in the hole!Theory of Hall Effect in a Two-Dimensional Electron SystemNew Method for High-Accuracy Determination of the Fine-Structure Constant Based on Quantized Hall ResistanceQuantized Hall conductivity in two dimensionsQuantization of particle transportQuasiadiabatic continuation of quantum states: The stability of topological ground-state degeneracy and emergent gauge invarianceQuasi-adiabatic Continuation of Quantum States: The Stability of Topological Ground State Degeneracy and Emergent Gauge InvarianceQuasi-adiabatic Continuation for Disordered Systems: Applications to Correlations, Lieb-Schultz-Mattis, and Hall ConductanceQuantization of Hall Conductance for Interacting Electrons on a TorusQuantization of Hall Conductance For Interacting Electrons Without Averaging AssumptionsHow Scientists Solved One of the Greatest Open Questions in Quantum PhysicsResonant Tunneling in the Quantum Hall Regime: Measurement of Fractional ChargeObservation of the e/3 Fractionally Charged Laughlin QuasiparticleObservation of the e/3 fractionally charged Laughlin quasiparticlesQuantum Spin Hall Effect in GrapheneQuantum Spin Hall Effect in GrapheneModel for a Quantum Hall Effect without Landau Levels: Condensed-Matter Realization of the "Parity Anomaly"Inflationary universe: A possible solution to the horizon and flatness problemsA New Type of Isotropic Cosmological Models Without SingularityDynamics of the Universe and Spontaneous Symmetry BreakingCosmological baryon-number domain structure and the first order phase transition of a vacuumMonopole production in the very early universe in a first-order phase transitionA new inflationary universe scenario: A possible solution of the horizon, flatness, homogeneity, isotropy and primordial monopole problemsCosmology for Grand Unified Theories with Radiatively Induced Symmetry BreakingQuantum fluctuations and a nonsingular universeVacuum energy and large-scale structure of the UniverseThe development of irregularities in a single bubble inflationary universeDynamics of Phase Transition in the New Inflationary Universe Scenario and Generation of PerturbationsFluctuations in the New Inflationary UniverseSpontaneous creation of almost scale-free density perturbations in an inflationary universeInflationary Predictions for Scalar and Tensor Fluctuations ReconsideredInflationary predictions for scalar and tensor fluctuations reconsideredCosmological constraints from the SDSS luminous red galaxiesCosmological Constraints from the SDSS Luminous Red GalaxiesCosmological Perturbations: Myths and FactsWhat does inflation really predict?What does inflation really predict?Planck 2015 results XIII. Cosmological parametersPlanck 2015 results XX. Constraints on inflationThree-Year Wilkinson Microwave Anisotropy Probe (WMAP) Observations: Implications for CosmologyDetection of B-Mode Polarization at Degree Angular Scales by BICEP2Planck intermediate results XXX. The angular power spectrum of polarized dust emission at intermediate and high Galactic latitudesPlanck intermediate results XXX. The angular power spectrum of polarized dust emission at intermediate and high Galactic latitudesFive years after BICEP2Inflationary paradigm in trouble after Planck2013Inflationary schism after Planck2013Implications of Planck2015 for inflationary, ekpyrotic and anamorphic bouncing cosmologiesSailing through the big crunch-big bang transitionCosmic Inflation Theory Faces ChallengesIntroduction to Early Universe CosmologyInfluence of Retardation on the London–van der Waals ForcesThe Influence of Retardation on the London-van der Waals ForcesThe Influence of Retardation on the London-van der Waals ForcesOn the attraction between two perfectly conducting plates.Casimir effect and the quantum vacuumThe Casimir Effect and the Quantum VacuumDemonstration of the Casimir Force in the 0.6 to 6 μm RangePrecision Measurement of the Casimir Force from 0.1 to 0.9 μmA Precision Measurement of the Casimir Force from 0.1 to 0.9 µmMeasurement of the Casimir Force between Parallel Metallic SurfacesMeasurement of the Casimir force between parallel metallic surfacesFrom Big Bang to Eternity?Late stages of crunchThe Big Crunch | MRS Bulletin | Cambridge CoreIndeterministic Quantum Gravity and Cosmology VII. Dynamical Passage through Singularities: Black Hole and Naked Singularity, Big Crunch and Big BangThe big crunchMeasurements of ω and λ from 42 High-Redshift SupernovaeA New Determination of the Hubble Constant from Globular Clusters in M87The Infall Velocity toward Virgo, the Hubble Constant, and a Search for Motion toward the Microwave BackgroundA Measurement of the Value of the Hubble Constant from the X-Ray Properties and the Sunyaev-Zel'Dovich Effect of Abell 665A Measurement of the Hubble Constant from the X-Ray Properties and the Sunyaev-Zel'Dovich Effect of Abell 2218The Case for a Hubble Constant of 30 km/s/MpcDetermining the Hubble Constant from the Gravitational Lens PG 1115+080The Dn-σ Relation for Bulges of Disk Galaxies: A New, Independent Measure of the Hubble ConstantThe Hubble Constant from Nickel Radioactivity in Type Ia SupernovaeLimits on the Hubble Constant from the HST Distance of M100A New Model of the Gravitational Lens 0957+561 and a Limit on the Hubble ConstantMeasurement of the Hubble Constant from X-Ray and 2.1 Millimeter Observations of Abell 2163The SBF Survey of Galaxy Distances. I. Sample Selection, Photometric Calibration, and the Hubble ConstantThe High-Z supernova Search: Measuring Cosmic Deceleration and Global Curvature of the Universe Using Type Ia SupernovaeThe Hubble Space Telescope Key Project on the Extragalactic Distance Scale. XV. A Cepheid Distance to the Fornax Cluster and its ImplicationsCepheid distance to M96 and the Hubble constantThe Hubble Space Telescope Key Project on the Extragalactic Distance Scale.XXVI. The Calibration of Population II Secondary Distance Indicators and the Value of the Hubble ConstantA Determination of the Hubble Constant from Cepheid Distances and a Model of the Local Peculiar Velocity FieldFinal Results from the Hubble Space Telescope Key Project to Measure the Hubble ConstantA New Cepheid Distance to the Maser-Host Galaxy NGC 4258 and its Implications for the Hubble ConstantCepheid Period-Luminosity Relations in the Near-Infrared and the Distance to M31 from the Hubble Space Telescope Wide Field Camera 3Optical Light Curve of the Type Ia Supernova 1998bu in M96 and the Supernova Calibration of the Hubble ConstantCepheid Calibrations from the Hubble Space Telescope of the Luminosity of Two Recent Type Ia Supernovae and a Redetermination of the Hubble ConstantA 3% solution: Determination of the Hubble Constant with the Hubble Space Telescope and Wide Field Camera 3Cosmic Microwave Background Observables and Their Cosmological ImplicationsCosmology from cosmic microwave background and galaxy clustersThe Age of the Universe and the Cosmological Constant Determined from Cosmic Microwave Background Anisotropy MeasurementsEvidence for a non-zero Λ and a low matter density from a combined analysis of the 2dF Galaxy Redshift Survey and cosmic microwave background anisotropiesA Measurement of the Cosmic Microwave Background Damping Tail from the 2500-Square-Degree SPT-SZ SurveyThe Hubble constant and the expansion age of the UniverseThe Hubble Constant from Gravitational Lens Time DelaysAnnual Review of Astronomy and Astrophysics: The Hubble ConstantMeasuring the Hubble constant with observations of water-vapor megamasersPlanck 2013 results. XVI. Cosmological parametersCarnegie Hubble Program: A Mid-Infrared Calibration of the Hubble ConstantHow a Dispute over a Single Number Became a Cosmological CrisisA 2.4% Determination Of The Local Value Of The Hubble ConstantNew Parallaxes of Galactic Cepheids from Spatially Scanning the Hubble Space Telescope: Implications for the Hubble ConstantPlanck 2018 results. VI. Cosmological parametersLarge Magellanic Cloud Cepheid Standards Provide a 1% Foundation for the Determination of the Hubble Constant and Stronger Evidence for Physics beyond ΛCDMThe Carnegie-Chicago Hubble Program. VIII. An Independent Determination of the Hubble Constant Based on the Tip of the Red Giant BranchConsistent Calibration of the Tip of the Red Giant Branch in the Large Magellanic Cloud on the Hubble Space Telescope Photometric System and a Redetermination of the Hubble ConstantThe Atacama Cosmology Telescope: DR4 Maps and Cosmological ParametersThe Atacama Cosmology Telescope: A Measurement of the Cosmic Microwave Background Power Spectra at 98 and 150 GHzMeasuring the Hubble constant with a sample of kilonovaePreposterous Universe: The dark energy equation of stateVacuum stabilityA phantom menace? Cosmological consequences of a dark energy component with super-negative equation of stateA Phantom Menace? Cosmological Consequences of a Dark Energy Component with Super-Negative Equation of StatePhantom Energy: Dark Energy with w<1 Causes a Cosmic DoomsdayThe End of Everything (Astrophysically Speaking)Mass of the Higgs BosonA Phenomenological Profile of the Higgs BosonHiggs Boson at the LHCObserving an invisible Higgs bosonHiggs Boson Theory and PhenomenologySearch for the Standard Model Higgs boson at LEPReview of Particle PhysicsObservation of Top Quark Production in ¯pp Collisions with the Collider Detector at FermilabObservation of Top Quark Production in ¯pp CollisionsObservation of the Top QuarkObservation of the Top QuarkUpdated Combination of CDF and D0 Searches for Standard Model Higgs Boson Production with up to 10.0 fb-1 of DataUnderlying event characteristics and their dependence on jet size of charged-particle jet events in p p collisions at √ ( s ) = 7 TeV with the ATLAS detectorSearch for supersymmetry in events with b -quark jets and missing transverse energy in p p collisions at 7 TeVCombined Search for the Standard Model Higgs Boson Decaying to a b¯b Pair Using the Full CDF Data SetCombined search for the standard model Higgs boson decaying to a bb pair using the full CDF data setCombined Search for the Standard Model Higgs Boson Decaying to b¯b Using the D0 Run II Data SetCombined search for the standard model Higgs boson decaying to b¯b using the D0 Run II data setEvidence for a Particle Produced in Association with Weak Bosons and Decaying to a Bottom-Antibottom Quark Pair in Higgs Boson Searches at the TevatronEvidence for a particle produced in association with weak bosons and decaying to a bottom-antibottom quark pair in Higgs boson searches at the TevatronObservation of a new particle in the search for the Standard Model Higgs boson with the ATLAS detector at the LHCObservation of a new boson at a mass of 125 GeV with the CMS experiment at the LHCConstraining anomalous Higgs boson interactionsHiggs boson mass and new physicsThe Higgs-boson lineshapeHeavy-quark mass effects in Higgs boson production at the LHCEvidence for the spin-0 nature of the Higgs boson using ATLAS dataNNLOPS simulation of Higgs boson productionInvestigating the near-criticality of the Higgs bosonMeasurement of the properties of a Higgs boson in the four-lepton final stateHiggs Boson Gluon-Fusion Production in QCD at Three LoopsLifetime and decay of "excited vacuum" states of a field theory associated with nonabsolute minima of its effective potentialSemiclassical methods for unstable statesConsequences of vacuum instability in quantum field theoryFate of the false vacuum: Semiclassical theoryFate of the false vacuum: Semiclassical theoryFate of the false vacuum. II. First quantum correctionsVacuum Instability and Higgs Scalar MassInflationary universe: A possible solution to the horizon and flatness problemsBounds on the Fermions and Higgs Boson Masses in Grand Unified TheoriesIs our vacuum metastable?False Vacuum: Early Universe Cosmology and the Development of InflationThe top quark and Higgs boson masses and the stability of the electroweak vacuumProbable or improbable universe? Correlating electroweak vacuum instability with the scale of inflationElectroweak vacuum instability and renormalized Higgs field vacuum fluctuations in the inflationary universeElectroweak Vacuum Instability and Renormalized Higgs Field Vacuum Fluctuations in the Inflationary UniverseElectroweak vacuum instability and renormalized vacuum field fluctuations in Friedmann-Lemaitre-Robertson-Walker backgroundThe probable fate of the Standard ModelHiggs boson and top quark masses as tests of electroweak vacuum stabilityHiggs boson and Top quark masses as tests of Electroweak Vacuum StabilityCosmological Aspects of Higgs Vacuum MetastabilityA simple motivated completion of the standard model below the Planck scale: Axions and right-handed neutrinosTop mass determination, Higgs inflation, and vacuum stabilityVacuum metastability with black holesPrimordial black hole formation by vacuum bubblesPrimordial black hole formation by vacuum bubblesVacuum decays around spinning black holesOn the Existence of n-Geodesically Complete or Future Complete Solutions of Einstein's Field Equations with Smooth Asymptotic StructureThe Global Nonlinear Stability of the Minkowski SpaceA proof of the instability of AdS for the Einstein--null dust system with an inner mirrorA proof of the instability of AdS for the Einstein--massless Vlasov systemBlack Holes Help Prove That a Special Kind of Space-Time Is UnstableGravitational effects on and of vacuum decayGravitational effects on and of vacuum decayThe End of Everything (Astrophysically Speaking)Observation of Gravitational Waves from a Binary Black Hole MergerGravitational Waves Discovered from Colliding Black HolesGW151226: Observation of Gravitational Waves from a 22-Solar-Mass Binary Black Hole CoalescenceGW170104: Observation of a 50-Solar-Mass Binary Black Hole Coalescence at Redshift 0.2GW170814: A Three-Detector Observation of Gravitational Waves from a Binary Black Hole CoalescenceGW170608: Observation of a 19-Solar-Mass Black Hole CoalescenceGWTC-1: A Gravitational-Wave Transient Catalog of Compact Binary Mergers Observed by LIGO and Virgo during the First and Second Observing RunsGW190412: Observation of a Binary-Black-Hole Coalescence with Asymmetric MassesGW170817: Observation of Gravitational Waves from a Binary Neutron Star InspiralGW190425: Observation of a Compact Binary Coalescence with Total Mass ∼ 3.4M Solar MassesGW190814: Gravitational Waves from the Coalescence of a 23 Solar Mass Black Hole with a 2.6 Solar Mass Compact ObjectMystery Object Blurs Line between Neutron Stars and Black HolesRelativistic Shapiro delay measurements of an extremely massive millisecond pulsarRelativistic Shapiro delay measurements of an extremely massive millisecond pulsarA noninteracting low-mass black hole–giant star binary systemDiscovery of a Candidate Black Hole−Giant Star Binary System in the Galactic FieldHypothesis concerning quark starsRemarks on quark starsStrange-Matter StarsGW190814: Impact of a 2.6 solar mass neutron star on nucleonic equations of stateLHCb Experiment Discovers CP Violation in the Charm Quark SystemLHCb discovers matter-antimatter asymmetry in charm quarksSearching for matter-antimatter asymmetry in the Higgs boson–top quark interactionNeutrino Asymmetry Passes Critical ThresholdClosing in on matter-antimatter asymmetry: T2K results restrict possible values of neutrino CP phasePostinflationary Higgs relaxation and the origin of matter-antimatter asymmetryA Strange New Higgs Particle May Have Stolen the Antimatter from Our UniverseHiggs Troika for Baryon AsymmetryEnd of inflation, oscillons and matter-antimatter asymmetryThe origin of dark matter, matter-anti-matter asymmetry, and inflationPrimordial black holes and the origin of the matter-antimatter asymmetryPrimordial Black Hole BaryogenesisPhysicists propose mechanism that explains the origins of both dark matter and 'normal' matterMatter-antimatter asymmetry and dark matter from torsionObservation of excess events in the XENON1T dark matter experimentDark Matter Experiment Finds Unexplained SignalSeeking Dark Matter, They Detected Another MysteryDark matter hunt yields unexplained signalPhysicists Announce Potential Dark Matter BreakthroughObservation of Excess Electronic Recoil Events in XENON1TIs Dark Matter Made of Axions?Case for axion origin of dark matter gains tractionAxion Kinetic Misalignment MechanismTheory and Phenomenology of CP ViolationCP Conservation in the Presence of PseudoparticlesAxions and Family Symmetry BreakingWeak-Interaction Singlet and Strong CP InvarianceCan confinement ensure natural CP invariance of strong interactions?A simple solution to the strong CP problem with a harmless axionCosmology of the Invisible AxionA cosmological bound on the invisible axionThe not-so-harmless axionCalculation of the axion mass based on high-temperature lattice quantum chromodynamicsSearch for axion-like dark matter with ferromagnetsSearch for axion-like dark matter with ferromagnetsEvidence Builds for a New Kind of NeutrinoAn Alternative Analysis of the LSND Neutrino Oscillation Search Data on ¯νμ→¯νeResults from the LSND Neutrino Oscillation Search for ¯νµ → ¯νeThe liquid scintillator neutrino detector and LAMPF neutrino sourceThe Liquid Scintillator Neutrino Detector and LAMPF Neutrino SourceSignificant Excess of Electronlike Events in the MiniBooNE Short-Baseline Neutrino ExperimentHidden Neutrino Particles May Be a Link to the Dark SectorSearch for GeV-Scale Sterile Neutrinos Responsible for Active Neutrino Oscillations and Baryon Asymmetry of the UniverseImproved Constraints on Sterile Neutrino Mixing from Disappearance Searches in the MINOS, MINOS+, Daya Bay, and Bugey-3 ExperimentsThe Black Hole Information Paradox Comes to an EndInformation in black hole radiationBlack Hole InformationIs Black-Hole Evaporation Predictable?Holographic Quantum Error Correction and the Projected Black Hole InteriorThe entropy of bulk quantum fields and the entanglement wedge of an evaporating black holeEntanglement Wedge Reconstruction and the Information ParadoxEvaporation of large black holes in AdS: coupling to the evaporonQuantum Extremal Surfaces: Holographic Entanglement Entropy beyond the Classical RegimeThe entropy of bulk quantum fields and the entanglement wedge of an evaporating black holeIslands in Schwarzschild black holesThe Page curve of Hawking radiation from semiclassical geometryHologram Within a Hologram Hints at Fate of Black HolesReplica wormholes and the black hole interiorReplica Wormholes and the Entropy of Hawking RadiationCool horizons for entangled black holesThe Universe from ScratchIn Violation of Einstein, Black Holes Might Have ‘Hair’Horizon Instability of Extremal Black HolesHorizon Hair of Extremal Black Holes and Measurements at Null InfinityScalar and gravitational hair for extreme Kerr black holesPhysics Beyond the Standard Model and Dark MatterBeyond the Standard ModelQuantum gravity: general introduction and recent developmentsQuantum Gravity: General Introduction and Recent DevelopmentsBlack Hole Singularities Are as Inescapable as ExpectedAxion-Induced Topology Change in Quantum Gravity and String TheoryNotes on Topological String Theory and 2d Quantum GravityFermionic string field theory of c = 1 two-dimensional quantum gravityA generalized uncertainty principle in quantum gravityA Generalized Uncertainty Principle in Quantum GravityAdS2 quantum gravity and string theoryLocality in quantum gravity and string theoryLocality in quantum gravity and string theoryQuantum gravity, dynamical phase-space and string theoryTwo-loop quantum gravityBlack Hole Entropy from Loop Quantum GravityBlack Hole Entropy from Loop Quantum Gravity“Sum over surfaces” form of loop quantum gravity"Sum over Surfaces" form of Loop Quantum GravityLoop quantum gravity and light propagationLoop quantum gravity and light propagationLectures on Loop Quantum GravityLectures on Loop Quantum GravityBlack-hole entropy in loop quantum gravityBlack hole entropy in Loop Quantum GravityLoop-Quantum-Gravity Vertex AmplitudeThe loop-quantum-gravity vertex-amplitudeLoop Quantum GravityPolyhedra in loop quantum gravityPolyhedra in loop quantum gravityUnity of All Elementary-Particle ForcesAspects of the grand unification of strong, weak and electromagnetic interactionsAspects of the Grand Unification of Strong, Weak and Electromagnetic InteractionsTheory of EverythingWMAP- Fate of the UniverseSpace and time in the quantum universe.Thermodynamics in the viscous early universeThermodynamics in the Viscous Early UniverseThermodynamics of viscous matter and radiation in the early universeThermodynamics of viscous Matter and Radiation in the Early universeIs emergent universe a consequence of particle creation process?Structure of Relatively Accelerating UniverseFootnotes[1] http://prints.iiap.res.in/bitstream/2248/4044/1/Evolution%20of%20the%20Universe%20through%20the%20Planck%20epoch[1] http://prints.iiap.res.in/bitstream/2248/4044/1/Evolution%20of%20the%20Universe%20through%20the%20Planck%20epoch[1] http://prints.iiap.res.in/bitstream/2248/4044/1/Evolution%20of%20the%20Universe%20through%20the%20Planck%20epoch[1] http://prints.iiap.res.in/bitstream/2248/4044/1/Evolution%20of%20the%20Universe%20through%20the%20Planck%20epoch[2] Cosmic Evolution - Epoch 1 - Particle Evolution[2] Cosmic Evolution - Epoch 1 - Particle Evolution[2] Cosmic Evolution - Epoch 1 - Particle Evolution[2] Cosmic Evolution - Epoch 1 - Particle Evolution[3] https://arxiv.org/pdf/hep-ph/0604270.pdf[3] https://arxiv.org/pdf/hep-ph/0604270.pdf[3] https://arxiv.org/pdf/hep-ph/0604270.pdf[3] https://arxiv.org/pdf/hep-ph/0604270.pdf[4] https://arxiv.org/pdf/1909.01820.pdf[4] https://arxiv.org/pdf/1909.01820.pdf[4] https://arxiv.org/pdf/1909.01820.pdf[4] https://arxiv.org/pdf/1909.01820.pdf[5] https://www.annualreviews.org/doi/pdf/10.1146/annurev.nucl.46.1.71[5] https://www.annualreviews.org/doi/pdf/10.1146/annurev.nucl.46.1.71[5] https://www.annualreviews.org/doi/pdf/10.1146/annurev.nucl.46.1.71[5] https://www.annualreviews.org/doi/pdf/10.1146/annurev.nucl.46.1.71[6] https://arxiv.org/pdf/nucl-th/0305030.pdf[6] https://arxiv.org/pdf/nucl-th/0305030.pdf[6] https://arxiv.org/pdf/nucl-th/0305030.pdf[6] https://arxiv.org/pdf/nucl-th/0305030.pdf[7] 1984ApJ...282..370B Page 370[7] 1984ApJ...282..370B Page 370[7] 1984ApJ...282..370B Page 370[7] 1984ApJ...282..370B Page 370[8] https://escholarship.org/content/qt9qb0f33k/qt9qb0f33k.pdf[8] https://escholarship.org/content/qt9qb0f33k/qt9qb0f33k.pdf[8] https://escholarship.org/content/qt9qb0f33k/qt9qb0f33k.pdf[8] https://escholarship.org/content/qt9qb0f33k/qt9qb0f33k.pdf[9] The matter-antimatter asymmetry problem[9] The matter-antimatter asymmetry problem[9] The matter-antimatter asymmetry problem[9] The matter-antimatter asymmetry problem[10] https://arxiv.org/pdf/astro-ph/9506015.pdf[10] https://arxiv.org/pdf/astro-ph/9506015.pdf[10] https://arxiv.org/pdf/astro-ph/9506015.pdf[10] https://arxiv.org/pdf/astro-ph/9506015.pdf[11] ShieldSquare Captcha[11] ShieldSquare Captcha[11] ShieldSquare Captcha[11] ShieldSquare Captcha[12] https://arxiv.org/pdf/astro-ph/9803095.pdf[12] https://arxiv.org/pdf/astro-ph/9803095.pdf[12] https://arxiv.org/pdf/astro-ph/9803095.pdf[12] https://arxiv.org/pdf/astro-ph/9803095.pdf[13] Constraints on the cosmic neutrino background[13] Constraints on the cosmic neutrino background[13] Constraints on the cosmic neutrino background[13] Constraints on the cosmic neutrino background[14] https://arxiv.org/pdf/1503.07863.pdf[14] https://arxiv.org/pdf/1503.07863.pdf[14] https://arxiv.org/pdf/1503.07863.pdf[14] https://arxiv.org/pdf/1503.07863.pdf[15] Baryogenesis via leptogenesis[15] Baryogenesis via leptogenesis[15] Baryogenesis via leptogenesis[15] Baryogenesis via leptogenesis[16] https://arxiv.org/pdf/hep-ph/0309342.pdf[16] https://arxiv.org/pdf/hep-ph/0309342.pdf[16] https://arxiv.org/pdf/hep-ph/0309342.pdf[16] https://arxiv.org/pdf/hep-ph/0309342.pdf[17] https://www.annualreviews.org/doi/pdf/10.1146/annurev.nucl.55.090704.151558[17] https://www.annualreviews.org/doi/pdf/10.1146/annurev.nucl.55.090704.151558[17] https://www.annualreviews.org/doi/pdf/10.1146/annurev.nucl.55.090704.151558[17] https://www.annualreviews.org/doi/pdf/10.1146/annurev.nucl.55.090704.151558[18] https://arxiv.org/pdf/0802.2962.pdf[18] https://arxiv.org/pdf/0802.2962.pdf[18] https://arxiv.org/pdf/0802.2962.pdf[18] https://arxiv.org/pdf/0802.2962.pdf[19] 1973ApJ...179..343W Page 343[19] 1973ApJ...179..343W Page 343[19] 1973ApJ...179..343W Page 343[19] 1973ApJ...179..343W Page 343[20] https://ntrs.nasa.gov/archive/nasa/casi.ntrs.nasa.gov/19900013110.pdf[20] https://ntrs.nasa.gov/archive/nasa/casi.ntrs.nasa.gov/19900013110.pdf[20] https://ntrs.nasa.gov/archive/nasa/casi.ntrs.nasa.gov/19900013110.pdf[20] https://ntrs.nasa.gov/archive/nasa/casi.ntrs.nasa.gov/19900013110.pdf[21] 1991ApJ...376...51W Page 51[21] 1991ApJ...376...51W Page 51[21] 1991ApJ...376...51W Page 51[21] 1991ApJ...376...51W Page 51[22] https://arxiv.org/pdf/astro-ph/9905320.pdf[22] https://arxiv.org/pdf/astro-ph/9905320.pdf[22] https://arxiv.org/pdf/astro-ph/9905320.pdf[22] https://arxiv.org/pdf/astro-ph/9905320.pdf[23] Planck and the cosmic microwave background[23] Planck and the cosmic microwave background[23] Planck and the cosmic microwave background[23] Planck and the cosmic microwave background[24] https://www.scientificamerican.com/article/the-dark-ages-of-the-univ-2006-11/[24] https://www.scientificamerican.com/article/the-dark-ages-of-the-univ-2006-11/[24] https://www.scientificamerican.com/article/the-dark-ages-of-the-univ-2006-11/[24] https://www.scientificamerican.com/article/the-dark-ages-of-the-univ-2006-11/[25] https://arxiv.org/pdf/0905.0929.pdf[25] https://arxiv.org/pdf/0905.0929.pdf[25] https://arxiv.org/pdf/0905.0929.pdf[25] https://arxiv.org/pdf/0905.0929.pdf[26] https://iopscience.iop.org/article/10.1086/383612/pdf[26] https://iopscience.iop.org/article/10.1086/383612/pdf[26] https://iopscience.iop.org/article/10.1086/383612/pdf[26] https://iopscience.iop.org/article/10.1086/383612/pdf[27] Dark Energy | Definition of Dark Energy by Oxford Dictionary on Lexico.com also meaning of Dark Energy[27] Dark Energy | Definition of Dark Energy by Oxford Dictionary on Lexico.com also meaning of Dark Energy[27] Dark Energy | Definition of Dark Energy by Oxford Dictionary on Lexico.com also meaning of Dark Energy[27] Dark Energy | Definition of Dark Energy by Oxford Dictionary on Lexico.com also meaning of Dark Energy[28] https://www.maths.tcd.ie/pub/HistMath/People/Newton/Principia/Bk1Sect1/PrBk1St1.pdf[28] https://www.maths.tcd.ie/pub/HistMath/People/Newton/Principia/Bk1Sect1/PrBk1St1.pdf[28] https://www.maths.tcd.ie/pub/HistMath/People/Newton/Principia/Bk1Sect1/PrBk1St1.pdf[28] https://www.maths.tcd.ie/pub/HistMath/People/Newton/Principia/Bk1Sect1/PrBk1St1.pdf[29] II. The Bakerian Lecture. On the theory of light and colours[29] II. The Bakerian Lecture. On the theory of light and colours[29] II. The Bakerian Lecture. On the theory of light and colours[29] II. The Bakerian Lecture. On the theory of light and colours[30] Biodiversity Heritage Library[30] Biodiversity Heritage Library[30] Biodiversity Heritage Library[30] Biodiversity Heritage Library[31] Biodiversity Heritage Library[31] Biodiversity Heritage Library[31] Biodiversity Heritage Library[31] Biodiversity Heritage Library[32] Weitere Studien über das Wärmegleichgewicht unter Gasmolekülen[32] Weitere Studien über das Wärmegleichgewicht unter Gasmolekülen[32] Weitere Studien über das Wärmegleichgewicht unter Gasmolekülen[32] Weitere Studien über das Wärmegleichgewicht unter Gasmolekülen[33] http://users.polytech.unice.fr/~leroux/boltztrad.pdf[33] http://users.polytech.unice.fr/~leroux/boltztrad.pdf[33] http://users.polytech.unice.fr/~leroux/boltztrad.pdf[33] http://users.polytech.unice.fr/~leroux/boltztrad.pdf[34] http://crystal.med.upenn.edu/sharp-lab-pdfs/2015SharpMatschinsky_Boltz1877_Entropy17.pdf[34] http://crystal.med.upenn.edu/sharp-lab-pdfs/2015SharpMatschinsky_Boltz1877_Entropy17.pdf[34] http://crystal.med.upenn.edu/sharp-lab-pdfs/2015SharpMatschinsky_Boltz1877_Entropy17.pdf[34] http://crystal.med.upenn.edu/sharp-lab-pdfs/2015SharpMatschinsky_Boltz1877_Entropy17.pdf[35] https://www.jstor.org/stable/pdf/112081.pdf[35] https://www.jstor.org/stable/pdf/112081.pdf[35] https://www.jstor.org/stable/pdf/112081.pdf[35] https://www.jstor.org/stable/pdf/112081.pdf[36] https://www.jstor.org/stable/pdf/108892.pdf[36] https://www.jstor.org/stable/pdf/108892.pdf[36] https://www.jstor.org/stable/pdf/108892.pdf[36] https://www.jstor.org/stable/pdf/108892.pdf[37] https://www.jstor.org/stable/2369245?origin=crossref&seq=1[37] https://www.jstor.org/stable/2369245?origin=crossref&seq=1[37] https://www.jstor.org/stable/2369245?origin=crossref&seq=1[37] https://www.jstor.org/stable/2369245?origin=crossref&seq=1[38] https://www.jstor.org/stable/pdf/2369245.pdf[38] https://www.jstor.org/stable/pdf/2369245.pdf[38] https://www.jstor.org/stable/pdf/2369245.pdf[38] https://www.jstor.org/stable/pdf/2369245.pdf[39] https://web.mit.edu/8.13/8.13c/references-fall/relativisticdynamics/thomson-cathode-rays-1897.pdf[39] https://web.mit.edu/8.13/8.13c/references-fall/relativisticdynamics/thomson-cathode-rays-1897.pdf[39] https://web.mit.edu/8.13/8.13c/references-fall/relativisticdynamics/thomson-cathode-rays-1897.pdf[39] https://web.mit.edu/8.13/8.13c/references-fall/relativisticdynamics/thomson-cathode-rays-1897.pdf[40] The American journal of science : Free Download, Borrow, and Streaming : Internet Archive[40] The American journal of science : Free Download, Borrow, and Streaming : Internet Archive[40] The American journal of science : Free Download, Borrow, and Streaming : Internet Archive[40] The American journal of science : Free Download, Borrow, and Streaming : Internet Archive[41] 1887SidM....6..306M Page 306[41] 1887SidM....6..306M Page 306[41] 1887SidM....6..306M Page 306[41] 1887SidM....6..306M Page 306[42] Lorentz Invariance Still Stands[42] Lorentz Invariance Still Stands[42] Lorentz Invariance Still Stands[42] Lorentz Invariance Still Stands[43] Constraints on Lorentz Invariance Violation from HAWC Observations of Gamma Rays above 100 TeV[43] Constraints on Lorentz Invariance Violation from HAWC Observations of Gamma Rays above 100 TeV[43] Constraints on Lorentz Invariance Violation from HAWC Observations of Gamma Rays above 100 TeV[43] Constraints on Lorentz Invariance Violation from HAWC Observations of Gamma Rays above 100 TeV[44] https://arxiv.org/pdf/1911.08070.pdf[44] https://arxiv.org/pdf/1911.08070.pdf[44] https://arxiv.org/pdf/1911.08070.pdf[44] https://arxiv.org/pdf/1911.08070.pdf[45] Physicists Have Tested The Speed of Light at The Highest Energies Yet[45] Physicists Have Tested The Speed of Light at The Highest Energies Yet[45] Physicists Have Tested The Speed of Light at The Highest Energies Yet[45] Physicists Have Tested The Speed of Light at The Highest Energies Yet[46] http://hermes.ffn.ub.es/luisnavarro/nuevo_maletin/Planck%20(1900),%20Distribution%20Law.pdf[46] http://hermes.ffn.ub.es/luisnavarro/nuevo_maletin/Planck%20(1900),%20Distribution%20Law.pdf[46] http://hermes.ffn.ub.es/luisnavarro/nuevo_maletin/Planck%20(1900),%20Distribution%20Law.pdf[46] http://hermes.ffn.ub.es/luisnavarro/nuevo_maletin/Planck%20(1900),%20Distribution%20Law.pdf[47] https://www.zbp.univie.ac.at/dokumente/einstein1.pdf[47] https://www.zbp.univie.ac.at/dokumente/einstein1.pdf[47] https://www.zbp.univie.ac.at/dokumente/einstein1.pdf[47] https://www.zbp.univie.ac.at/dokumente/einstein1.pdf[48] http://users.physik.fu-berlin.de/~kleinert/files/eins_lq.pdf[48] http://users.physik.fu-berlin.de/~kleinert/files/eins_lq.pdf[48] http://users.physik.fu-berlin.de/~kleinert/files/eins_lq.pdf[48] http://users.physik.fu-berlin.de/~kleinert/files/eins_lq.pdf[49] http://chiuphysics.cgu.edu.tw/yun-ju/cguweb/PhyChiu/H305Relativity/EinsteinSpecialRelativity.pdf[49] http://chiuphysics.cgu.edu.tw/yun-ju/cguweb/PhyChiu/H305Relativity/EinsteinSpecialRelativity.pdf[49] http://chiuphysics.cgu.edu.tw/yun-ju/cguweb/PhyChiu/H305Relativity/EinsteinSpecialRelativity.pdf[49] http://chiuphysics.cgu.edu.tw/yun-ju/cguweb/PhyChiu/H305Relativity/EinsteinSpecialRelativity.pdf[50] http://www.astro.puc.cl/~rparra/tools/PAPERS/e_mc2.pdf[50] http://www.astro.puc.cl/~rparra/tools/PAPERS/e_mc2.pdf[50] http://www.astro.puc.cl/~rparra/tools/PAPERS/e_mc2.pdf[50] http://www.astro.puc.cl/~rparra/tools/PAPERS/e_mc2.pdf[51] http://hermes.ffn.ub.es/luisnavarro/nuevo_maletin/Einstein_GRelativity_1916.pdf[51] http://hermes.ffn.ub.es/luisnavarro/nuevo_maletin/Einstein_GRelativity_1916.pdf[51] http://hermes.ffn.ub.es/luisnavarro/nuevo_maletin/Einstein_GRelativity_1916.pdf[51] http://hermes.ffn.ub.es/luisnavarro/nuevo_maletin/Einstein_GRelativity_1916.pdf[52] http://newt.phys.unsw.edu.au/~jkw/phys3550/GWs/Einstein_Rosen.pdf[52] http://newt.phys.unsw.edu.au/~jkw/phys3550/GWs/Einstein_Rosen.pdf[52] http://newt.phys.unsw.edu.au/~jkw/phys3550/GWs/Einstein_Rosen.pdf[52] http://newt.phys.unsw.edu.au/~jkw/phys3550/GWs/Einstein_Rosen.pdf[53] http://old.phys.huji.ac.il/~barak_kol/Courses/Black-holes/reading-papers/Einstein1939.pdf[53] http://old.phys.huji.ac.il/~barak_kol/Courses/Black-holes/reading-papers/Einstein1939.pdf[53] http://old.phys.huji.ac.il/~barak_kol/Courses/Black-holes/reading-papers/Einstein1939.pdf[53] http://old.phys.huji.ac.il/~barak_kol/Courses/Black-holes/reading-papers/Einstein1939.pdf[54] http://www.ymambrini.com/My_World/History_files/Eddington.pdf[54] http://www.ymambrini.com/My_World/History_files/Eddington.pdf[54] http://www.ymambrini.com/My_World/History_files/Eddington.pdf[54] http://www.ymambrini.com/My_World/History_files/Eddington.pdf[55] 1919Obs....42..119E Page 119[55] 1919Obs....42..119E Page 119[55] 1919Obs....42..119E Page 119[55] 1919Obs....42..119E Page 119[56] http://aflb.ensmp.fr/LDB-oeuvres/De_Broglie_Kracklauer.pdf[56] http://aflb.ensmp.fr/LDB-oeuvres/De_Broglie_Kracklauer.pdf[56] http://aflb.ensmp.fr/LDB-oeuvres/De_Broglie_Kracklauer.pdf[56] http://aflb.ensmp.fr/LDB-oeuvres/De_Broglie_Kracklauer.pdf[57] https://www.asc.ohio-state.edu/perry.6/p5500_au16/articles/broglie-lecture.pdf[57] https://www.asc.ohio-state.edu/perry.6/p5500_au16/articles/broglie-lecture.pdf[57] https://www.asc.ohio-state.edu/perry.6/p5500_au16/articles/broglie-lecture.pdf[57] https://www.asc.ohio-state.edu/perry.6/p5500_au16/articles/broglie-lecture.pdf[58] Diffraction of Cathode Rays by a Thin Film[58] Diffraction of Cathode Rays by a Thin Film[58] Diffraction of Cathode Rays by a Thin Film[58] Diffraction of Cathode Rays by a Thin Film[59] Reflection of Electrons by a Crystal of Nickel[59] Reflection of Electrons by a Crystal of Nickel[59] Reflection of Electrons by a Crystal of Nickel[59] Reflection of Electrons by a Crystal of Nickel[60] Elektroneninterferenzen an mehreren künstlich hergestellten Feinspalten[60] Elektroneninterferenzen an mehreren künstlich hergestellten Feinspalten[60] Elektroneninterferenzen an mehreren künstlich hergestellten Feinspalten[60] Elektroneninterferenzen an mehreren künstlich hergestellten Feinspalten[61] The Young-Feynman two-slits experiment with single electrons: Build-up of the interference pattern and arrival-time distribution using a fast-readout pixel detector[61] The Young-Feynman two-slits experiment with single electrons: Build-up of the interference pattern and arrival-time distribution using a fast-readout pixel detector[61] The Young-Feynman two-slits experiment with single electrons: Build-up of the interference pattern and arrival-time distribution using a fast-readout pixel detector[61] The Young-Feynman two-slits experiment with single electrons: Build-up of the interference pattern and arrival-time distribution using a fast-readout pixel detector[62] https://www.equipes.lps.u-psud.fr/Montambaux/histoire-physique/Bose-1924.pdf[62] https://www.equipes.lps.u-psud.fr/Montambaux/histoire-physique/Bose-1924.pdf[62] https://www.equipes.lps.u-psud.fr/Montambaux/histoire-physique/Bose-1924.pdf[62] https://www.equipes.lps.u-psud.fr/Montambaux/histoire-physique/Bose-1924.pdf[63] Plancks Gesetz und Lichtquantenhypothese[63] Plancks Gesetz und Lichtquantenhypothese[63] Plancks Gesetz und Lichtquantenhypothese[63] Plancks Gesetz und Lichtquantenhypothese[64] 1994JApA...15....3B Page 3[64] 1994JApA...15....3B Page 3[64] 1994JApA...15....3B Page 3[64] 1994JApA...15....3B Page 3[65] https://www.equipes.lps.u-psud.fr/Montambaux/histoire-physique/Pauli-1925-anglais.pdf[65] https://www.equipes.lps.u-psud.fr/Montambaux/histoire-physique/Pauli-1925-anglais.pdf[65] https://www.equipes.lps.u-psud.fr/Montambaux/histoire-physique/Pauli-1925-anglais.pdf[65] https://www.equipes.lps.u-psud.fr/Montambaux/histoire-physique/Pauli-1925-anglais.pdf[66] http://web.ihep.su/dbserv/compas/src/pauli40b/eng.pdf[66] http://web.ihep.su/dbserv/compas/src/pauli40b/eng.pdf[66] http://web.ihep.su/dbserv/compas/src/pauli40b/eng.pdf[66] http://web.ihep.su/dbserv/compas/src/pauli40b/eng.pdf[67] http://users.mat.unimi.it/users/galgani/arch/heis25ajp.pdf[67] http://users.mat.unimi.it/users/galgani/arch/heis25ajp.pdf[67] http://users.mat.unimi.it/users/galgani/arch/heis25ajp.pdf[67] http://users.mat.unimi.it/users/galgani/arch/heis25ajp.pdf[68] http://www.neo-classical-physics.info/uploads/3/4/3/6/34363841/born_and_jordan_-_qm_1.pdf[68] http://www.neo-classical-physics.info/uploads/3/4/3/6/34363841/born_and_jordan_-_qm_1.pdf[68] http://www.neo-classical-physics.info/uploads/3/4/3/6/34363841/born_and_jordan_-_qm_1.pdf[68] http://www.neo-classical-physics.info/uploads/3/4/3/6/34363841/born_and_jordan_-_qm_1.pdf[69] Über das Wasserstoffspektrum vom Standpunkt der neuen Quantenmechanik[69] Über das Wasserstoffspektrum vom Standpunkt der neuen Quantenmechanik[69] Über das Wasserstoffspektrum vom Standpunkt der neuen Quantenmechanik[69] Über das Wasserstoffspektrum vom Standpunkt der neuen Quantenmechanik[70] Quantisierung als Eigenwertproblem[70] Quantisierung als Eigenwertproblem[70] Quantisierung als Eigenwertproblem[70] Quantisierung als Eigenwertproblem[71] http://www.physics.drexel.edu/~bob/Quantum_Papers/Schr_1.pdf[71] http://www.physics.drexel.edu/~bob/Quantum_Papers/Schr_1.pdf[71] http://www.physics.drexel.edu/~bob/Quantum_Papers/Schr_1.pdf[71] http://www.physics.drexel.edu/~bob/Quantum_Papers/Schr_1.pdf[72] http://materias.df.uba.ar/f4Aa2012c2/files/2012/08/schrod.pdf[72] http://materias.df.uba.ar/f4Aa2012c2/files/2012/08/schrod.pdf[72] http://materias.df.uba.ar/f4Aa2012c2/files/2012/08/schrod.pdf[72] http://materias.df.uba.ar/f4Aa2012c2/files/2012/08/schrod.pdf[73] Zur Quantenmechanik der Stoßvorgänge[73] Zur Quantenmechanik der Stoßvorgänge[73] Zur Quantenmechanik der Stoßvorgänge[73] Zur Quantenmechanik der Stoßvorgänge[74] http://www.neo-classical-physics.info/uploads/3/0/6/5/3065888/born_-_qm_for_collisions_ii.pdf[74] http://www.neo-classical-physics.info/uploads/3/0/6/5/3065888/born_-_qm_for_collisions_ii.pdf[74] http://www.neo-classical-physics.info/uploads/3/0/6/5/3065888/born_-_qm_for_collisions_ii.pdf[74] http://www.neo-classical-physics.info/uploads/3/0/6/5/3065888/born_-_qm_for_collisions_ii.pdf[75] https://arxiv.org/pdf/cond-mat/9912229.pdf[75] https://arxiv.org/pdf/cond-mat/9912229.pdf[75] https://arxiv.org/pdf/cond-mat/9912229.pdf[75] https://arxiv.org/pdf/cond-mat/9912229.pdf[76] On the theory of quantum mechanics[76] On the theory of quantum mechanics[76] On the theory of quantum mechanics[76] On the theory of quantum mechanics[77] Über den anschaulichen Inhalt der quantentheoretischen Kinematik und Mechanik[77] Über den anschaulichen Inhalt der quantentheoretischen Kinematik und Mechanik[77] Über den anschaulichen Inhalt der quantentheoretischen Kinematik und Mechanik[77] Über den anschaulichen Inhalt der quantentheoretischen Kinematik und Mechanik[78] Zur Quantenmechanik einfacher Bewegungstypen[78] Zur Quantenmechanik einfacher Bewegungstypen[78] Zur Quantenmechanik einfacher Bewegungstypen[78] Zur Quantenmechanik einfacher Bewegungstypen[79] http://wwwhome.lorentz.leidenuniv.nl/~boyarsky/media/Proc.R.Soc.Lond.-1927-Dirac-243-65.pdf[79] http://wwwhome.lorentz.leidenuniv.nl/~boyarsky/media/Proc.R.Soc.Lond.-1927-Dirac-243-65.pdf[79] http://wwwhome.lorentz.leidenuniv.nl/~boyarsky/media/Proc.R.Soc.Lond.-1927-Dirac-243-65.pdf[79] http://wwwhome.lorentz.leidenuniv.nl/~boyarsky/media/Proc.R.Soc.Lond.-1927-Dirac-243-65.pdf[80] The quantum theory of the emission and absorption of radiation[80] The quantum theory of the emission and absorption of radiation[80] The quantum theory of the emission and absorption of radiation[80] The quantum theory of the emission and absorption of radiation[81] http://neo-classical-physics.info/uploads/3/4/3/6/34363841/jordan_and_pauli_-_qed_of_charge-free_fields.pdf[81] http://neo-classical-physics.info/uploads/3/4/3/6/34363841/jordan_and_pauli_-_qed_of_charge-free_fields.pdf[81] http://neo-classical-physics.info/uploads/3/4/3/6/34363841/jordan_and_pauli_-_qed_of_charge-free_fields.pdf[81] http://neo-classical-physics.info/uploads/3/4/3/6/34363841/jordan_and_pauli_-_qed_of_charge-free_fields.pdf[82] Zur Quantenelektrodynamik ladungsfreier Felder[82] Zur Quantenelektrodynamik ladungsfreier Felder[82] Zur Quantenelektrodynamik ladungsfreier Felder[82] Zur Quantenelektrodynamik ladungsfreier Felder[83] http://fafnir.phyast.pitt.edu/py3765/FermiQED.pdf[83] http://fafnir.phyast.pitt.edu/py3765/FermiQED.pdf[83] http://fafnir.phyast.pitt.edu/py3765/FermiQED.pdf[83] http://fafnir.phyast.pitt.edu/py3765/FermiQED.pdf[84] Quantum Theory of Radiation[84] Quantum Theory of Radiation[84] Quantum Theory of Radiation[84] Quantum Theory of Radiation[85] https://arxiv.org/pdf/physics/0605038.pdf[85] https://arxiv.org/pdf/physics/0605038.pdf[85] https://arxiv.org/pdf/physics/0605038.pdf[85] https://arxiv.org/pdf/physics/0605038.pdf[86] Folgerungen aus der Diracschen Theorie des Positrons[86] Folgerungen aus der Diracschen Theorie des Positrons[86] Folgerungen aus der Diracschen Theorie des Positrons[86] Folgerungen aus der Diracschen Theorie des Positrons[87] http://cdsads.u-strasbg.fr/cgi-bin/nph-iarticle_query?1927ASSB...47...49L&data_type=PDF_HIGH&whole_paper=YES&type=PRINTER&filetype=.pdf[87] http://cdsads.u-strasbg.fr/cgi-bin/nph-iarticle_query?1927ASSB...47...49L&data_type=PDF_HIGH&whole_paper=YES&type=PRINTER&filetype=.pdf[87] http://cdsads.u-strasbg.fr/cgi-bin/nph-iarticle_query?1927ASSB...47...49L&data_type=PDF_HIGH&whole_paper=YES&type=PRINTER&filetype=.pdf[87] http://cdsads.u-strasbg.fr/cgi-bin/nph-iarticle_query?1927ASSB...47...49L&data_type=PDF_HIGH&whole_paper=YES&type=PRINTER&filetype=.pdf[88] Homogeneous Universe of Constant Mass and Increasing Radius accounting for the Radial Velocity of Extra-galactic Nebulæ[88] Homogeneous Universe of Constant Mass and Increasing Radius accounting for the Radial Velocity of Extra-galactic Nebulæ[88] Homogeneous Universe of Constant Mass and Increasing Radius accounting for the Radial Velocity of Extra-galactic Nebulæ[88] Homogeneous Universe of Constant Mass and Increasing Radius accounting for the Radial Velocity of Extra-galactic Nebulæ[89] The Beginning of the World from the Point of View of Quantum Theory[89] The Beginning of the World from the Point of View of Quantum Theory[89] The Beginning of the World from the Point of View of Quantum Theory[89] The Beginning of the World from the Point of View of Quantum Theory[90] http://banburytuition.coffeecup.com/Physics%20A%20Level/Quantum%20mechanics/Images%20and%20documents/The%20Quantum%20Theory%20of%20the%20Electron%20Dirac.pdf[90] http://banburytuition.coffeecup.com/Physics%20A%20Level/Quantum%20mechanics/Images%20and%20documents/The%20Quantum%20Theory%20of%20the%20Electron%20Dirac.pdf[90] http://banburytuition.coffeecup.com/Physics%20A%20Level/Quantum%20mechanics/Images%20and%20documents/The%20Quantum%20Theory%20of%20the%20Electron%20Dirac.pdf[90] http://banburytuition.coffeecup.com/Physics%20A%20Level/Quantum%20mechanics/Images%20and%20documents/The%20Quantum%20Theory%20of%20the%20Electron%20Dirac.pdf[91] http://www.weylmann.com/Part%20II.pdf[91] http://www.weylmann.com/Part%20II.pdf[91] http://www.weylmann.com/Part%20II.pdf[91] http://www.weylmann.com/Part%20II.pdf[92] https://journals.aps.org/pr/pdf/10.1103/PhysRev.43.491[92] https://journals.aps.org/pr/pdf/10.1103/PhysRev.43.491[92] https://journals.aps.org/pr/pdf/10.1103/PhysRev.43.491[92] https://journals.aps.org/pr/pdf/10.1103/PhysRev.43.491[93] https://www.pnas.org/content/pnas/15/3/168.full.pdf[93] https://www.pnas.org/content/pnas/15/3/168.full.pdf[93] https://www.pnas.org/content/pnas/15/3/168.full.pdf[93] https://www.pnas.org/content/pnas/15/3/168.full.pdf[94] https://www.nature.com/articles/132406a0.pdf?origin=ppub[94] https://www.nature.com/articles/132406a0.pdf?origin=ppub[94] https://www.nature.com/articles/132406a0.pdf?origin=ppub[94] https://www.nature.com/articles/132406a0.pdf?origin=ppub[95] The Expanding Universe[95] The Expanding Universe[95] The Expanding Universe[95] The Expanding Universe[96] The Large Numbers hypothesis and the Einstein theory of gravitation[96] The Large Numbers hypothesis and the Einstein theory of gravitation[96] The Large Numbers hypothesis and the Einstein theory of gravitation[96] The Large Numbers hypothesis and the Einstein theory of gravitation[97] http://calteches.library.caltech.edu/3684/1/Universe.pdf[97] http://calteches.library.caltech.edu/3684/1/Universe.pdf[97] http://calteches.library.caltech.edu/3684/1/Universe.pdf[97] http://calteches.library.caltech.edu/3684/1/Universe.pdf[98] 1975ApJ...196..313S Page 313[98] 1975ApJ...196..313S Page 313[98] 1975ApJ...196..313S Page 313[98] 1975ApJ...196..313S Page 313[99] 1975ApJ...197..265S Page 265[99] 1975ApJ...197..265S Page 265[99] 1975ApJ...197..265S Page 265[99] 1975ApJ...197..265S Page 265[100] 1982ApJ...256..339S Page 339[100] 1982ApJ...256..339S Page 339[100] 1982ApJ...256..339S Page 339[100] 1982ApJ...256..339S Page 339[101] Origin of the Hubble constant controversy[101] Origin of the Hubble constant controversy[101] Origin of the Hubble constant controversy[101] Origin of the Hubble constant controversy[102] 1991ApJ...373L...1T Page L1[102] 1991ApJ...373L...1T Page L1[102] 1991ApJ...373L...1T Page L1[102] 1991ApJ...373L...1T Page L1[103] http://www.sternwarte.uni-erlangen.de/~drechsel/vorlesungen/physiksterne/HD/cepheid/nature_pierce.pdf[103] http://www.sternwarte.uni-erlangen.de/~drechsel/vorlesungen/physiksterne/HD/cepheid/nature_pierce.pdf[103] http://www.sternwarte.uni-erlangen.de/~drechsel/vorlesungen/physiksterne/HD/cepheid/nature_pierce.pdf[103] http://www.sternwarte.uni-erlangen.de/~drechsel/vorlesungen/physiksterne/HD/cepheid/nature_pierce.pdf[104] https://arxiv.org/pdf/astro-ph/9410054.pdf[104] https://arxiv.org/pdf/astro-ph/9410054.pdf[104] https://arxiv.org/pdf/astro-ph/9410054.pdf[104] https://arxiv.org/pdf/astro-ph/9410054.pdf[105] https://arxiv.org/pdf/astro-ph/9509160.pdf[105] https://arxiv.org/pdf/astro-ph/9509160.pdf[105] https://arxiv.org/pdf/astro-ph/9509160.pdf[105] https://arxiv.org/pdf/astro-ph/9509160.pdf[106] 1995AJ....110.1476K Page 1476[106] 1995AJ....110.1476K Page 1476[106] 1995AJ....110.1476K Page 1476[106] 1995AJ....110.1476K Page 1476[107] http://www.ifsc.usp.br/~laf/quantica2/dirac_monopole_original_paper_1931.pdf[107] http://www.ifsc.usp.br/~laf/quantica2/dirac_monopole_original_paper_1931.pdf[107] http://www.ifsc.usp.br/~laf/quantica2/dirac_monopole_original_paper_1931.pdf[107] http://www.ifsc.usp.br/~laf/quantica2/dirac_monopole_original_paper_1931.pdf[108] Quantised singularities in the electromagnetic field,[108] Quantised singularities in the electromagnetic field,[108] Quantised singularities in the electromagnetic field,[108] Quantised singularities in the electromagnetic field,[109] Evidence for Detection of a Moving Magnetic Monopole[109] Evidence for Detection of a Moving Magnetic Monopole[109] Evidence for Detection of a Moving Magnetic Monopole[109] Evidence for Detection of a Moving Magnetic Monopole[110] First Results from a Superconductive Detector for Moving Magnetic Monopoles[110] First Results from a Superconductive Detector for Moving Magnetic Monopoles[110] First Results from a Superconductive Detector for Moving Magnetic Monopoles[110] First Results from a Superconductive Detector for Moving Magnetic Monopoles[111] https://arxiv.org/pdf/hep-ex/0602040.pdf[111] https://arxiv.org/pdf/hep-ex/0602040.pdf[111] https://arxiv.org/pdf/hep-ex/0602040.pdf[111] https://arxiv.org/pdf/hep-ex/0602040.pdf[112] https://authors.library.caltech.edu/6133/1/PREprl79.pdf[112] https://authors.library.caltech.edu/6133/1/PREprl79.pdf[112] https://authors.library.caltech.edu/6133/1/PREprl79.pdf[112] https://authors.library.caltech.edu/6133/1/PREprl79.pdf[113] https://repository.ust.hk/ir/bitstream/1783.1-49322/1/PhysRevLett.44.631.pdf[113] https://repository.ust.hk/ir/bitstream/1783.1-49322/1/PhysRevLett.44.631.pdf[113] https://repository.ust.hk/ir/bitstream/1783.1-49322/1/PhysRevLett.44.631.pdf[113] https://repository.ust.hk/ir/bitstream/1783.1-49322/1/PhysRevLett.44.631.pdf[114] Supercooled phase transitions in the very early universe[114] Supercooled phase transitions in the very early universe[114] Supercooled phase transitions in the very early universe[114] Supercooled phase transitions in the very early universe[115] https://www.annualreviews.org/doi/pdf/10.1146/annurev.ns.34.120184.002333[115] https://www.annualreviews.org/doi/pdf/10.1146/annurev.ns.34.120184.002333[115] https://www.annualreviews.org/doi/pdf/10.1146/annurev.ns.34.120184.002333[115] https://www.annualreviews.org/doi/pdf/10.1146/annurev.ns.34.120184.002333[116] 1933AcHPh...6..110Z Page 110[116] 1933AcHPh...6..110Z Page 110[116] 1933AcHPh...6..110Z Page 110[116] 1933AcHPh...6..110Z Page 110[117] http://www.ymambrini.com/My_World/History_files/Zwicky.pdf[117] http://www.ymambrini.com/My_World/History_files/Zwicky.pdf[117] http://www.ymambrini.com/My_World/History_files/Zwicky.pdf[117] http://www.ymambrini.com/My_World/History_files/Zwicky.pdf[118] 1937ApJ....86..217Z Page 217[118] 1937ApJ....86..217Z Page 217[118] 1937ApJ....86..217Z Page 217[118] 1937ApJ....86..217Z Page 217[119] https://journals.aps.org/pr/pdf/10.1103/PhysRev.47.777[119] https://journals.aps.org/pr/pdf/10.1103/PhysRev.47.777[119] https://journals.aps.org/pr/pdf/10.1103/PhysRev.47.777[119] https://journals.aps.org/pr/pdf/10.1103/PhysRev.47.777[120] https://journals.aps.org/pr/pdf/10.1103/PhysRev.48.696[120] https://journals.aps.org/pr/pdf/10.1103/PhysRev.48.696[120] https://journals.aps.org/pr/pdf/10.1103/PhysRev.48.696[120] https://journals.aps.org/pr/pdf/10.1103/PhysRev.48.696[121] https://www.informationphilosopher.com/solutions/scientists/einstein/Physik_und_Realitat.pdf[121] https://www.informationphilosopher.com/solutions/scientists/einstein/Physik_und_Realitat.pdf[121] https://www.informationphilosopher.com/solutions/scientists/einstein/Physik_und_Realitat.pdf[121] https://www.informationphilosopher.com/solutions/scientists/einstein/Physik_und_Realitat.pdf[122] Physics and reality[122] Physics and reality[122] Physics and reality[122] Physics and reality[123] http://ursula.chem.yale.edu/~batista/classes/v572/Bohm1.pdf[123] http://ursula.chem.yale.edu/~batista/classes/v572/Bohm1.pdf[123] http://ursula.chem.yale.edu/~batista/classes/v572/Bohm1.pdf[123] http://ursula.chem.yale.edu/~batista/classes/v572/Bohm1.pdf[124] http://physics.nmsu.edu/~bkiefer/HISTORY/BOHM_1952.pdf[124] http://physics.nmsu.edu/~bkiefer/HISTORY/BOHM_1952.pdf[124] http://physics.nmsu.edu/~bkiefer/HISTORY/BOHM_1952.pdf[124] http://physics.nmsu.edu/~bkiefer/HISTORY/BOHM_1952.pdf[125] https://www.informationphilosopher.com/solutions/scientists/schrodinger/Die_Situation-3.pdf[125] https://www.informationphilosopher.com/solutions/scientists/schrodinger/Die_Situation-3.pdf[125] https://www.informationphilosopher.com/solutions/scientists/schrodinger/Die_Situation-3.pdf[125] https://www.informationphilosopher.com/solutions/scientists/schrodinger/Die_Situation-3.pdf[126] http://hermes.ffn.ub.es/luisnavarro/nuevo_maletin/Schrodinger_1935_cat.pdf[126] http://hermes.ffn.ub.es/luisnavarro/nuevo_maletin/Schrodinger_1935_cat.pdf[126] http://hermes.ffn.ub.es/luisnavarro/nuevo_maletin/Schrodinger_1935_cat.pdf[126] http://hermes.ffn.ub.es/luisnavarro/nuevo_maletin/Schrodinger_1935_cat.pdf[127] https://journals.aps.org/pr/pdf/10.1103/PhysRev.73.803[127] https://journals.aps.org/pr/pdf/10.1103/PhysRev.73.803[127] https://journals.aps.org/pr/pdf/10.1103/PhysRev.73.803[127] https://journals.aps.org/pr/pdf/10.1103/PhysRev.73.803[128] Note on the Theory of the Interaction of Field and Matter[128] Note on the Theory of the Interaction of Field and Matter[128] Note on the Theory of the Interaction of Field and Matter[128] Note on the Theory of the Interaction of Field and Matter[129] Note on the Radiation Field of the Electron[129] Note on the Radiation Field of the Electron[129] Note on the Radiation Field of the Electron[129] Note on the Radiation Field of the Electron[130] On the Self-Energy and the Electromagnetic Field of the Electron[130] On the Self-Energy and the Electromagnetic Field of the Electron[130] On the Self-Energy and the Electromagnetic Field of the Electron[130] On the Self-Energy and the Electromagnetic Field of the Electron[131] https://journals.aps.org/pr/pdf/10.1103/PhysRev.72.241[131] https://journals.aps.org/pr/pdf/10.1103/PhysRev.72.241[131] https://journals.aps.org/pr/pdf/10.1103/PhysRev.72.241[131] https://journals.aps.org/pr/pdf/10.1103/PhysRev.72.241[132] On the Intrinsic Moment of the Electron[132] On the Intrinsic Moment of the Electron[132] On the Intrinsic Moment of the Electron[132] On the Intrinsic Moment of the Electron[133] The Electromagnetic Shift of Energy Levels[133] The Electromagnetic Shift of Energy Levels[133] The Electromagnetic Shift of Energy Levels[133] The Electromagnetic Shift of Energy Levels[134] On a Relativistically Invariant Formulation of the Quantum Theory of Wave Fields.[134] On a Relativistically Invariant Formulation of the Quantum Theory of Wave Fields.[134] On a Relativistically Invariant Formulation of the Quantum Theory of Wave Fields.[134] On a Relativistically Invariant Formulation of the Quantum Theory of Wave Fields.[135] https://journals.aps.org/pr/pdf/10.1103/PhysRev.73.416[135] https://journals.aps.org/pr/pdf/10.1103/PhysRev.73.416[135] https://journals.aps.org/pr/pdf/10.1103/PhysRev.73.416[135] https://journals.aps.org/pr/pdf/10.1103/PhysRev.73.416[136] Quantum Electrodynamics. I. A Covariant Formulation[136] Quantum Electrodynamics. I. A Covariant Formulation[136] Quantum Electrodynamics. I. A Covariant Formulation[136] Quantum Electrodynamics. I. A Covariant Formulation[137] Quantum Electrodynamics. II. Vacuum Polarization and Self-Energy[137] Quantum Electrodynamics. II. Vacuum Polarization and Self-Energy[137] Quantum Electrodynamics. II. Vacuum Polarization and Self-Energy[137] Quantum Electrodynamics. II. Vacuum Polarization and Self-Energy[138] Quantum Electrodynamics. III. The Electromagnetic Properties of the Electron---Radiative Corrections to Scattering[138] Quantum Electrodynamics. III. The Electromagnetic Properties of the Electron---Radiative Corrections to Scattering[138] Quantum Electrodynamics. III. The Electromagnetic Properties of the Electron---Radiative Corrections to Scattering[138] Quantum Electrodynamics. III. The Electromagnetic Properties of the Electron---Radiative Corrections to Scattering[139] https://journals.aps.org/pr/pdf/10.1103/PhysRev.75.486[139] https://journals.aps.org/pr/pdf/10.1103/PhysRev.75.486[139] https://journals.aps.org/pr/pdf/10.1103/PhysRev.75.486[139] https://journals.aps.org/pr/pdf/10.1103/PhysRev.75.486[140] The $S$ Matrix in Quantum Electrodynamics[140] The $S$ Matrix in Quantum Electrodynamics[140] The $S$ Matrix in Quantum Electrodynamics[140] The $S$ Matrix in Quantum Electrodynamics[141] The Theory of Positrons[141] The Theory of Positrons[141] The Theory of Positrons[141] The Theory of Positrons[142] https://journals.aps.org/pr/pdf/10.1103/PhysRev.76.769[142] https://journals.aps.org/pr/pdf/10.1103/PhysRev.76.769[142] https://journals.aps.org/pr/pdf/10.1103/PhysRev.76.769[142] https://journals.aps.org/pr/pdf/10.1103/PhysRev.76.769[143] Mathematical Formulation of the Quantum Theory of Electromagnetic Interaction[143] Mathematical Formulation of the Quantum Theory of Electromagnetic Interaction[143] Mathematical Formulation of the Quantum Theory of Electromagnetic Interaction[143] Mathematical Formulation of the Quantum Theory of Electromagnetic Interaction[144] Über den Bau der Atomkerne. I[144] Über den Bau der Atomkerne. I[144] Über den Bau der Atomkerne. I[144] Über den Bau der Atomkerne. I[145] On the Consequences of the Symmetry of the Nuclear Hamiltonian on the Spectroscopy of Nuclei[145] On the Consequences of the Symmetry of the Nuclear Hamiltonian on the Spectroscopy of Nuclei[145] On the Consequences of the Symmetry of the Nuclear Hamiltonian on the Spectroscopy of Nuclei[145] On the Consequences of the Symmetry of the Nuclear Hamiltonian on the Spectroscopy of Nuclei[146] Charge Independence for V -particles * [146] Charge Independence for V -particles * [146] Charge Independence for V -particles * [146] Charge Independence for V -particles * [147] Charge Independence Theory of V Particles * [147] Charge Independence Theory of V Particles * [147] Charge Independence Theory of V Particles * [147] Charge Independence Theory of V Particles * [148] https://pdfs.semanticscholar.org/2cda/266f4d9dcf5086563612051638b007463ff4.pdf[148] https://pdfs.semanticscholar.org/2cda/266f4d9dcf5086563612051638b007463ff4.pdf[148] https://pdfs.semanticscholar.org/2cda/266f4d9dcf5086563612051638b007463ff4.pdf[148] https://pdfs.semanticscholar.org/2cda/266f4d9dcf5086563612051638b007463ff4.pdf[149] https://journals.aps.org/rmp/pdf/10.1103/RevModPhys.29.547[149] https://journals.aps.org/rmp/pdf/10.1103/RevModPhys.29.547[149] https://journals.aps.org/rmp/pdf/10.1103/RevModPhys.29.547[149] https://journals.aps.org/rmp/pdf/10.1103/RevModPhys.29.547[150] 1954ApJS....1..121H Page 121[150] 1954ApJS....1..121H Page 121[150] 1954ApJS....1..121H Page 121[150] 1954ApJS....1..121H Page 121[151] The Synthesis of the Elements from Hydrogen ⋆ [151] The Synthesis of the Elements from Hydrogen ⋆ [151] The Synthesis of the Elements from Hydrogen ⋆ [151] The Synthesis of the Elements from Hydrogen ⋆ [152] https://journals.aps.org/pr/pdf/10.1103/PhysRev.55.434[152] https://journals.aps.org/pr/pdf/10.1103/PhysRev.55.434[152] https://journals.aps.org/pr/pdf/10.1103/PhysRev.55.434[152] https://journals.aps.org/pr/pdf/10.1103/PhysRev.55.434[153] https://fas.org/sgp/eprint/CRL-41.pdf[153] https://fas.org/sgp/eprint/CRL-41.pdf[153] https://fas.org/sgp/eprint/CRL-41.pdf[153] https://fas.org/sgp/eprint/CRL-41.pdf[154] Neutron capture chains in heavy element synthesis[154] Neutron capture chains in heavy element synthesis[154] Neutron capture chains in heavy element synthesis[154] Neutron capture chains in heavy element synthesis[155] Nucleosynthesis of Heavy Elements by Neutron Capture.[155] Nucleosynthesis of Heavy Elements by Neutron Capture.[155] Nucleosynthesis of Heavy Elements by Neutron Capture.[155] Nucleosynthesis of Heavy Elements by Neutron Capture.[156] Nucleosynthesis During Silicon Burning[156] Nucleosynthesis During Silicon Burning[156] Nucleosynthesis During Silicon Burning[156] Nucleosynthesis During Silicon Burning[157] http://articles.adsabs.harvard.edu/pdf/1968ApJS...16..299B[157] http://articles.adsabs.harvard.edu/pdf/1968ApJS...16..299B[157] http://articles.adsabs.harvard.edu/pdf/1968ApJS...16..299B[157] http://articles.adsabs.harvard.edu/pdf/1968ApJS...16..299B[158] https://journals.aps.org/pr/pdf/10.1103/PhysRev.104.254[158] https://journals.aps.org/pr/pdf/10.1103/PhysRev.104.254[158] https://journals.aps.org/pr/pdf/10.1103/PhysRev.104.254[158] https://journals.aps.org/pr/pdf/10.1103/PhysRev.104.254[159] https://journals.aps.org/pr/pdf/10.1103/PhysRev.105.1413[159] https://journals.aps.org/pr/pdf/10.1103/PhysRev.105.1413[159] https://journals.aps.org/pr/pdf/10.1103/PhysRev.105.1413[159] https://journals.aps.org/pr/pdf/10.1103/PhysRev.105.1413[160] https://timelinefy-space-001.nyc3.digitaloceanspaces.com/files/8/8_D9RMKI0M4O7H82TRLHGQ3ESZWH07YFA2.pdf[160] https://timelinefy-space-001.nyc3.digitaloceanspaces.com/files/8/8_D9RMKI0M4O7H82TRLHGQ3ESZWH07YFA2.pdf[160] https://timelinefy-space-001.nyc3.digitaloceanspaces.com/files/8/8_D9RMKI0M4O7H82TRLHGQ3ESZWH07YFA2.pdf[160] https://timelinefy-space-001.nyc3.digitaloceanspaces.com/files/8/8_D9RMKI0M4O7H82TRLHGQ3ESZWH07YFA2.pdf[161] https://timelinefy-space-001.nyc3.digitaloceanspaces.com/files/8/8_08PAUGY3FL4VEPIAL6FVG8UPEULY9TTG.pdf[161] https://timelinefy-space-001.nyc3.digitaloceanspaces.com/files/8/8_08PAUGY3FL4VEPIAL6FVG8UPEULY9TTG.pdf[161] https://timelinefy-space-001.nyc3.digitaloceanspaces.com/files/8/8_08PAUGY3FL4VEPIAL6FVG8UPEULY9TTG.pdf[161] https://timelinefy-space-001.nyc3.digitaloceanspaces.com/files/8/8_08PAUGY3FL4VEPIAL6FVG8UPEULY9TTG.pdf[162] THE EIGHTFOLD WAY: A THEORY OF STRONG INTERACTION SYMMETRY (Technical Report)[162] THE EIGHTFOLD WAY: A THEORY OF STRONG INTERACTION SYMMETRY (Technical Report)[162] THE EIGHTFOLD WAY: A THEORY OF STRONG INTERACTION SYMMETRY (Technical Report)[162] THE EIGHTFOLD WAY: A THEORY OF STRONG INTERACTION SYMMETRY (Technical Report)[163] https://hep.caltech.edu/gm/images/quarks.pdf[163] https://hep.caltech.edu/gm/images/quarks.pdf[163] https://hep.caltech.edu/gm/images/quarks.pdf[163] https://hep.caltech.edu/gm/images/quarks.pdf[164] http://cds.cern.ch/record/352337/files/CERN-TH-401.pdf[164] http://cds.cern.ch/record/352337/files/CERN-TH-401.pdf[164] http://cds.cern.ch/record/352337/files/CERN-TH-401.pdf[164] http://cds.cern.ch/record/352337/files/CERN-TH-401.pdf[165] http://cds.cern.ch/record/570209/files/CERN-TH-412.pdf[165] http://cds.cern.ch/record/570209/files/CERN-TH-412.pdf[165] http://cds.cern.ch/record/570209/files/CERN-TH-412.pdf[165] http://cds.cern.ch/record/570209/files/CERN-TH-412.pdf[166] Magnetic moments of baryons in a quark model[166] Magnetic moments of baryons in a quark model[166] Magnetic moments of baryons in a quark model[166] Magnetic moments of baryons in a quark model[167] https://arxiv.org/ftp/arxiv/papers/0904/0904.0343.pdf[167] https://arxiv.org/ftp/arxiv/papers/0904/0904.0343.pdf[167] https://arxiv.org/ftp/arxiv/papers/0904/0904.0343.pdf[167] https://arxiv.org/ftp/arxiv/papers/0904/0904.0343.pdf[168] Spin and Unitary-Spin Independence in a Paraquark Model of Baryons and Mesons[168] Spin and Unitary-Spin Independence in a Paraquark Model of Baryons and Mesons[168] Spin and Unitary-Spin Independence in a Paraquark Model of Baryons and Mesons[168] Spin and Unitary-Spin Independence in a Paraquark Model of Baryons and Mesons[169] Three-Triplet Model with Double $\mathrm{SU}(3)$ Symmetry[169] Three-Triplet Model with Double $\mathrm{SU}(3)$ Symmetry[169] Three-Triplet Model with Double $\mathrm{SU}(3)$ Symmetry[169] Three-Triplet Model with Double $\mathrm{SU}(3)$ Symmetry[170] Plasmons, Gauge Invariance, and Mass[170] Plasmons, Gauge Invariance, and Mass[170] Plasmons, Gauge Invariance, and Mass[170] Plasmons, Gauge Invariance, and Mass[171] https://journals.aps.org/prl/pdf/10.1103/PhysRevLett.13.321[171] https://journals.aps.org/prl/pdf/10.1103/PhysRevLett.13.321[171] https://journals.aps.org/prl/pdf/10.1103/PhysRevLett.13.321[171] https://journals.aps.org/prl/pdf/10.1103/PhysRevLett.13.321[172] https://journals.aps.org/prl/pdf/10.1103/PhysRevLett.13.508[172] https://journals.aps.org/prl/pdf/10.1103/PhysRevLett.13.508[172] https://journals.aps.org/prl/pdf/10.1103/PhysRevLett.13.508[172] https://journals.aps.org/prl/pdf/10.1103/PhysRevLett.13.508[173] https://journals.aps.org/prl/pdf/10.1103/PhysRevLett.13.585[173] https://journals.aps.org/prl/pdf/10.1103/PhysRevLett.13.585[173] https://journals.aps.org/prl/pdf/10.1103/PhysRevLett.13.585[173] https://journals.aps.org/prl/pdf/10.1103/PhysRevLett.13.585[174] https://cds.cern.ch/record/343400/files/CM-P00057225.pdf?version=1&origin=publicationDetail[174] https://cds.cern.ch/record/343400/files/CM-P00057225.pdf?version=1&origin=publicationDetail[174] https://cds.cern.ch/record/343400/files/CM-P00057225.pdf?version=1&origin=publicationDetail[174] https://cds.cern.ch/record/343400/files/CM-P00057225.pdf?version=1&origin=publicationDetail[175] https://journals.aps.org/ppf/pdf/10.1103/PhysicsPhysiqueFizika.1.195[175] https://journals.aps.org/ppf/pdf/10.1103/PhysicsPhysiqueFizika.1.195[175] https://journals.aps.org/ppf/pdf/10.1103/PhysicsPhysiqueFizika.1.195[175] https://journals.aps.org/ppf/pdf/10.1103/PhysicsPhysiqueFizika.1.195[176] https://arxiv.org/pdf/quant-ph/0701097.pdf[176] https://arxiv.org/pdf/quant-ph/0701097.pdf[176] https://arxiv.org/pdf/quant-ph/0701097.pdf[176] https://arxiv.org/pdf/quant-ph/0701097.pdf[177] https://arxiv.org/pdf/0908.3408.pdf[177] https://arxiv.org/pdf/0908.3408.pdf[177] https://arxiv.org/pdf/0908.3408.pdf[177] https://arxiv.org/pdf/0908.3408.pdf[178] https://is.muni.cz/el/1441/podzim2017/FY2BP_TF1/um/Uceni_text_-_John_S._Bell_Speakable_and_Unspeakable_in_Quantum_Mechanics__First_Edition.pdf[178] https://is.muni.cz/el/1441/podzim2017/FY2BP_TF1/um/Uceni_text_-_John_S._Bell_Speakable_and_Unspeakable_in_Quantum_Mechanics__First_Edition.pdf[178] https://is.muni.cz/el/1441/podzim2017/FY2BP_TF1/um/Uceni_text_-_John_S._Bell_Speakable_and_Unspeakable_in_Quantum_Mechanics__First_Edition.pdf[178] https://is.muni.cz/el/1441/podzim2017/FY2BP_TF1/um/Uceni_text_-_John_S._Bell_Speakable_and_Unspeakable_in_Quantum_Mechanics__First_Edition.pdf[179] https://escholarship.org/content/qt2f18n5nk/qt2f18n5nk.pdf?t=p2au19[179] https://escholarship.org/content/qt2f18n5nk/qt2f18n5nk.pdf?t=p2au19[179] https://escholarship.org/content/qt2f18n5nk/qt2f18n5nk.pdf?t=p2au19[179] https://escholarship.org/content/qt2f18n5nk/qt2f18n5nk.pdf?t=p2au19[180] https://journals.aps.org/prl/pdf/10.1103/PhysRevLett.47.460[180] https://journals.aps.org/prl/pdf/10.1103/PhysRevLett.47.460[180] https://journals.aps.org/prl/pdf/10.1103/PhysRevLett.47.460[180] https://journals.aps.org/prl/pdf/10.1103/PhysRevLett.47.460[181] https://journals.aps.org/prl/pdf/10.1103/PhysRevLett.49.1804[181] https://journals.aps.org/prl/pdf/10.1103/PhysRevLett.49.1804[181] https://journals.aps.org/prl/pdf/10.1103/PhysRevLett.49.1804[181] https://journals.aps.org/prl/pdf/10.1103/PhysRevLett.49.1804[182] Experimental demonstration of quantum correlations over more than 10 km[182] Experimental demonstration of quantum correlations over more than 10 km[182] Experimental demonstration of quantum correlations over more than 10 km[182] Experimental demonstration of quantum correlations over more than 10 km[183] Violation of Bell Inequalities by Photons More Than 10 km Apart[183] Violation of Bell Inequalities by Photons More Than 10 km Apart[183] Violation of Bell Inequalities by Photons More Than 10 km Apart[183] Violation of Bell Inequalities by Photons More Than 10 km Apart[184] Violation of Bell's Inequality under Strict Einstein Locality Conditions[184] Violation of Bell's Inequality under Strict Einstein Locality Conditions[184] Violation of Bell's Inequality under Strict Einstein Locality Conditions[184] Violation of Bell's Inequality under Strict Einstein Locality Conditions[185] https://www.nature.com/articles/35000514[185] https://www.nature.com/articles/35000514[185] https://www.nature.com/articles/35000514[185] https://www.nature.com/articles/35000514[186] Experimental violation of a Bell's inequality with efficient detection[186] Experimental violation of a Bell's inequality with efficient detection[186] Experimental violation of a Bell's inequality with efficient detection[186] Experimental violation of a Bell's inequality with efficient detection[187] https://deepblue.lib.umich.edu/bitstream/handle/2027.42/62731/409791a0.pdf;jsessionid=4C0CB9B5EF9C09FEE4B9467E2898A953?sequence=1https://deepblue.lib.umich.edu/bitstream/handle/2027.42/62731/409791a0.pdf;jsessionid=4C0CB9B5EF9C09FEE4B9467E2898A953?sequence=1[187] https://deepblue.lib.umich.edu/bitstream/handle/2027.42/62731/409791a0.pdf;jsessionid=4C0CB9B5EF9C09FEE4B9467E2898A953?sequence=1https://deepblue.lib.umich.edu/bitstream/handle/2027.42/62731/409791a0.pdf;jsessionid=4C0CB9B5EF9C09FEE4B9467E2898A953?sequence=1[187] https://deepblue.lib.umich.edu/bitstream/handle/2027.42/62731/409791a0.pdf;jsessionid=4C0CB9B5EF9C09FEE4B9467E2898A953?sequence=1https://deepblue.lib.umich.edu/bitstream/handle/2027.42/62731/409791a0.pdf;jsessionid=4C0CB9B5EF9C09FEE4B9467E2898A953?sequence=1[187] https://deepblue.lib.umich.edu/bitstream/handle/2027.42/62731/409791a0.pdf;jsessionid=4C0CB9B5EF9C09FEE4B9467E2898A953?sequence=1https://deepblue.lib.umich.edu/bitstream/handle/2027.42/62731/409791a0.pdf;jsessionid=4C0CB9B5EF9C09FEE4B9467E2898A953?sequence=1[188] https://www.nature.com/articles/nature05677[188] https://www.nature.com/articles/nature05677[188] https://www.nature.com/articles/nature05677[188] https://www.nature.com/articles/nature05677[189] Erratum: An experimental test of non-local realism[189] Erratum: An experimental test of non-local realism[189] Erratum: An experimental test of non-local realism[189] Erratum: An experimental test of non-local realism[190] https://arxiv.org/pdf/0704.2529.pdf[190] https://arxiv.org/pdf/0704.2529.pdf[190] https://arxiv.org/pdf/0704.2529.pdf[190] https://arxiv.org/pdf/0704.2529.pdf[191] Spacelike Separation in a Bell Test Assuming Gravitationally Induced Collapses[191] Spacelike Separation in a Bell Test Assuming Gravitationally Induced Collapses[191] Spacelike Separation in a Bell Test Assuming Gravitationally Induced Collapses[191] Spacelike Separation in a Bell Test Assuming Gravitationally Induced Collapses[192] https://arxiv.org/pdf/0803.2425.pdf[192] https://arxiv.org/pdf/0803.2425.pdf[192] https://arxiv.org/pdf/0803.2425.pdf[192] https://arxiv.org/pdf/0803.2425.pdf[193] Violation of Bell's inequality in Josephson phase qubits[193] Violation of Bell's inequality in Josephson phase qubits[193] Violation of Bell's inequality in Josephson phase qubits[193] Violation of Bell's inequality in Josephson phase qubits[194] Bell violation using entangled photons without the fair-sampling assumption[194] Bell violation using entangled photons without the fair-sampling assumption[194] Bell violation using entangled photons without the fair-sampling assumption[194] Bell violation using entangled photons without the fair-sampling assumption[195] https://arxiv.org/ftp/arxiv/papers/1212/1212.0533.pdf[195] https://arxiv.org/ftp/arxiv/papers/1212/1212.0533.pdf[195] https://arxiv.org/ftp/arxiv/papers/1212/1212.0533.pdf[195] https://arxiv.org/ftp/arxiv/papers/1212/1212.0533.pdf[196] Detection-Loophole-Free Test of Quantum Nonlocality, and Applications[196] Detection-Loophole-Free Test of Quantum Nonlocality, and Applications[196] Detection-Loophole-Free Test of Quantum Nonlocality, and Applications[196] Detection-Loophole-Free Test of Quantum Nonlocality, and Applications[197] https://arxiv.org/pdf/1306.5772.pdf[197] https://arxiv.org/pdf/1306.5772.pdf[197] https://arxiv.org/pdf/1306.5772.pdf[197] https://arxiv.org/pdf/1306.5772.pdf[198] Bell-inequality violation with entangled photons, free of the coincidence-time loophole[198] Bell-inequality violation with entangled photons, free of the coincidence-time loophole[198] Bell-inequality violation with entangled photons, free of the coincidence-time loophole[198] Bell-inequality violation with entangled photons, free of the coincidence-time loophole[199] https://arxiv.org/pdf/1309.0712.pdf[199] https://arxiv.org/pdf/1309.0712.pdf[199] https://arxiv.org/pdf/1309.0712.pdf[199] https://arxiv.org/pdf/1309.0712.pdf[200] Loophole-free Bell inequality violation using electron spins separated by 1.3 kilometres[200] Loophole-free Bell inequality violation using electron spins separated by 1.3 kilometres[200] Loophole-free Bell inequality violation using electron spins separated by 1.3 kilometres[200] Loophole-free Bell inequality violation using electron spins separated by 1.3 kilometres[201] https://arxiv.org/pdf/1508.05949.pdf[201] https://arxiv.org/pdf/1508.05949.pdf[201] https://arxiv.org/pdf/1508.05949.pdf[201] https://arxiv.org/pdf/1508.05949.pdf[202] https://journals.aps.org/prl/pdf/10.1103/PhysRevLett.115.250401[202] https://journals.aps.org/prl/pdf/10.1103/PhysRevLett.115.250401[202] https://journals.aps.org/prl/pdf/10.1103/PhysRevLett.115.250401[202] https://journals.aps.org/prl/pdf/10.1103/PhysRevLett.115.250401[203] https://journals.aps.org/prl/pdf/10.1103/PhysRevLett.115.250402[203] https://journals.aps.org/prl/pdf/10.1103/PhysRevLett.115.250402[203] https://journals.aps.org/prl/pdf/10.1103/PhysRevLett.115.250402[203] https://journals.aps.org/prl/pdf/10.1103/PhysRevLett.115.250402[204] https://arxiv.org/pdf/1604.06419.pdf[204] https://arxiv.org/pdf/1604.06419.pdf[204] https://arxiv.org/pdf/1604.06419.pdf[204] https://arxiv.org/pdf/1604.06419.pdf[205] https://journals.aps.org/prl/pdf/10.1103/PhysRevLett.118.060401[205] https://journals.aps.org/prl/pdf/10.1103/PhysRevLett.118.060401[205] https://journals.aps.org/prl/pdf/10.1103/PhysRevLett.118.060401[205] https://journals.aps.org/prl/pdf/10.1103/PhysRevLett.118.060401[206] https://journals.aps.org/prl/pdf/10.1103/PhysRevLett.119.010402[206] https://journals.aps.org/prl/pdf/10.1103/PhysRevLett.119.010402[206] https://journals.aps.org/prl/pdf/10.1103/PhysRevLett.119.010402[206] https://journals.aps.org/prl/pdf/10.1103/PhysRevLett.119.010402[207] Challenging local realism with human choices[207] Challenging local realism with human choices[207] Challenging local realism with human choices[207] Challenging local realism with human choices[208] https://www.nature.com/articles/s41586-018-0489-0[208] https://www.nature.com/articles/s41586-018-0489-0[208] https://www.nature.com/articles/s41586-018-0489-0[208] https://www.nature.com/articles/s41586-018-0489-0[209] https://arxiv.org/pdf/1805.04431.pdf[209] https://arxiv.org/pdf/1805.04431.pdf[209] https://arxiv.org/pdf/1805.04431.pdf[209] https://arxiv.org/pdf/1805.04431.pdf[210] https://journals.aps.org/prl/pdf/10.1103/PhysRevLett.121.080403[210] https://journals.aps.org/prl/pdf/10.1103/PhysRevLett.121.080403[210] https://journals.aps.org/prl/pdf/10.1103/PhysRevLett.121.080403[210] https://journals.aps.org/prl/pdf/10.1103/PhysRevLett.121.080403[211] 1965ApJ...142..419P Page 419[211] 1965ApJ...142..419P Page 419[211] 1965ApJ...142..419P Page 419[211] 1965ApJ...142..419P Page 419[212] 1965ApJ...142..414D Page 414[212] 1965ApJ...142..414D Page 414[212] 1965ApJ...142..414D Page 414[212] 1965ApJ...142..414D Page 414[213] http://articles.adsabs.harvard.edu/pdf/1948MNRAS.108..372H[213] http://articles.adsabs.harvard.edu/pdf/1948MNRAS.108..372H[213] http://articles.adsabs.harvard.edu/pdf/1948MNRAS.108..372H[213] http://articles.adsabs.harvard.edu/pdf/1948MNRAS.108..372H[214] http://articles.adsabs.harvard.edu/pdf/1993ApJ...410..437H[214] http://articles.adsabs.harvard.edu/pdf/1993ApJ...410..437H[214] http://articles.adsabs.harvard.edu/pdf/1993ApJ...410..437H[214] http://articles.adsabs.harvard.edu/pdf/1993ApJ...410..437H[215] http://repository.iucaa.in:8080/jspui/bitstream/11007/1133/1/209A_1994.pdf[215] http://repository.iucaa.in:8080/jspui/bitstream/11007/1133/1/209A_1994.pdf[215] http://repository.iucaa.in:8080/jspui/bitstream/11007/1133/1/209A_1994.pdf[215] http://repository.iucaa.in:8080/jspui/bitstream/11007/1133/1/209A_1994.pdf[216] Erratum: Astrophysical deductions from the quasi-steady-state cosmology[216] Erratum: Astrophysical deductions from the quasi-steady-state cosmology[216] Erratum: Astrophysical deductions from the quasi-steady-state cosmology[216] Erratum: Astrophysical deductions from the quasi-steady-state cosmology[217] http://articles.adsabs.harvard.edu/pdf/1994A&A...289..729H[217] http://articles.adsabs.harvard.edu/pdf/1994A&A...289..729H[217] http://articles.adsabs.harvard.edu/pdf/1994A&A...289..729H[217] http://articles.adsabs.harvard.edu/pdf/1994A&A...289..729H[218] The basic theory underlying the quasi-steady-state cosmology[218] The basic theory underlying the quasi-steady-state cosmology[218] The basic theory underlying the quasi-steady-state cosmology[218] The basic theory underlying the quasi-steady-state cosmology[219] ShieldSquare Captcha[219] ShieldSquare Captcha[219] ShieldSquare Captcha[219] ShieldSquare Captcha[220] https://arxiv.org/pdf/astro-ph/9410070.pdf[220] https://arxiv.org/pdf/astro-ph/9410070.pdf[220] https://arxiv.org/pdf/astro-ph/9410070.pdf[220] https://arxiv.org/pdf/astro-ph/9410070.pdf[221] https://arxiv.org/pdf/astro-ph/9412045.pdf[221] https://arxiv.org/pdf/astro-ph/9412045.pdf[221] https://arxiv.org/pdf/astro-ph/9412045.pdf[221] https://arxiv.org/pdf/astro-ph/9412045.pdf[222] Errors in the Steady State and Quasi-SS Models[222] Errors in the Steady State and Quasi-SS Models[222] Errors in the Steady State and Quasi-SS Models[222] Errors in the Steady State and Quasi-SS Models[223] http://articles.adsabs.harvard.edu/pdf/1967ApJ...147...73S[223] http://articles.adsabs.harvard.edu/pdf/1967ApJ...147...73S[223] http://articles.adsabs.harvard.edu/pdf/1967ApJ...147...73S[223] http://articles.adsabs.harvard.edu/pdf/1967ApJ...147...73S[224] Large-scale Density Inhomogeneities in the Universe[224] Large-scale Density Inhomogeneities in the Universe[224] Large-scale Density Inhomogeneities in the Universe[224] Large-scale Density Inhomogeneities in the Universe[225] http://articles.adsabs.harvard.edu/pdf/1970Ap%26SS...7....3S[225] http://articles.adsabs.harvard.edu/pdf/1970Ap%26SS...7....3S[225] http://articles.adsabs.harvard.edu/pdf/1970Ap%26SS...7....3S[225] http://articles.adsabs.harvard.edu/pdf/1970Ap%26SS...7....3S[226] The Sunyaev–Zeldovich effect towards three clusters of galaxies[226] The Sunyaev–Zeldovich effect towards three clusters of galaxies[226] The Sunyaev–Zeldovich effect towards three clusters of galaxies[226] The Sunyaev–Zeldovich effect towards three clusters of galaxies[227] http://articles.adsabs.harvard.edu/pdf/1994ApJ...420..445F[227] http://articles.adsabs.harvard.edu/pdf/1994ApJ...420..445F[227] http://articles.adsabs.harvard.edu/pdf/1994ApJ...420..445F[227] http://articles.adsabs.harvard.edu/pdf/1994ApJ...420..445F[228] DMR Data Products[228] DMR Data Products[228] DMR Data Products[228] DMR Data Products[229] Detection of polarization in the cosmic microwave background using DASI[229] Detection of polarization in the cosmic microwave background using DASI[229] Detection of polarization in the cosmic microwave background using DASI[229] Detection of polarization in the cosmic microwave background using DASI[230] https://arxiv.org/pdf/astro-ph/0209478.pdf[230] https://arxiv.org/pdf/astro-ph/0209478.pdf[230] https://arxiv.org/pdf/astro-ph/0209478.pdf[230] https://arxiv.org/pdf/astro-ph/0209478.pdf[231] Polarization Observations with the Cosmic Background Imager[231] Polarization Observations with the Cosmic Background Imager[231] Polarization Observations with the Cosmic Background Imager[231] Polarization Observations with the Cosmic Background Imager[232] https://arxiv.org/pdf/astro-ph/0409569.pdf[232] https://arxiv.org/pdf/astro-ph/0409569.pdf[232] https://arxiv.org/pdf/astro-ph/0409569.pdf[232] https://arxiv.org/pdf/astro-ph/0409569.pdf[233] https://journals.aps.org/prl/pdf/10.1103/PhysRevLett.19.1264[233] https://journals.aps.org/prl/pdf/10.1103/PhysRevLett.19.1264[233] https://journals.aps.org/prl/pdf/10.1103/PhysRevLett.19.1264[233] https://journals.aps.org/prl/pdf/10.1103/PhysRevLett.19.1264[234] https://cds.cern.ch/record/417920/files/c74-07-01-p131.pdf[234] https://cds.cern.ch/record/417920/files/c74-07-01-p131.pdf[234] https://cds.cern.ch/record/417920/files/c74-07-01-p131.pdf[234] https://cds.cern.ch/record/417920/files/c74-07-01-p131.pdf[235] Experimental study of structure functions and sum rules in charge-changing interactions of neutrinos and antineutrinos on nucleons[235] Experimental study of structure functions and sum rules in charge-changing interactions of neutrinos and antineutrinos on nucleons[235] Experimental study of structure functions and sum rules in charge-changing interactions of neutrinos and antineutrinos on nucleons[235] Experimental study of structure functions and sum rules in charge-changing interactions of neutrinos and antineutrinos on nucleons[236] https://inis.iaea.org/collection/NCLCollectionStore/_Public/14/801/14801269.pdf[236] https://inis.iaea.org/collection/NCLCollectionStore/_Public/14/801/14801269.pdf[236] https://inis.iaea.org/collection/NCLCollectionStore/_Public/14/801/14801269.pdf[236] https://inis.iaea.org/collection/NCLCollectionStore/_Public/14/801/14801269.pdf[237] https://cds.cern.ch/record/182774/files/cer-000094196.pdf[237] https://cds.cern.ch/record/182774/files/cer-000094196.pdf[237] https://cds.cern.ch/record/182774/files/cer-000094196.pdf[237] https://cds.cern.ch/record/182774/files/cer-000094196.pdf[238] https://cds.cern.ch/record/2103277/files/9789814644150_0006.pdf[238] https://cds.cern.ch/record/2103277/files/9789814644150_0006.pdf[238] https://cds.cern.ch/record/2103277/files/9789814644150_0006.pdf[238] https://cds.cern.ch/record/2103277/files/9789814644150_0006.pdf[239] Event Horizons in Static Vacuum Space-Times[239] Event Horizons in Static Vacuum Space-Times[239] Event Horizons in Static Vacuum Space-Times[239] Event Horizons in Static Vacuum Space-Times[240] Event horizons in static electrovac space-times[240] Event horizons in static electrovac space-times[240] Event horizons in static electrovac space-times[240] Event horizons in static electrovac space-times[241] Axisymmetric Black Hole Has Only Two Degrees of Freedom[241] Axisymmetric Black Hole Has Only Two Degrees of Freedom[241] Axisymmetric Black Hole Has Only Two Degrees of Freedom[241] Axisymmetric Black Hole Has Only Two Degrees of Freedom[242] Gravitation[242] Gravitation[242] Gravitation[242] Gravitation[243] Geons[243] Geons[243] Geons[243] Geons[244] 1970ApJ...159..379R Page 379[244] 1970ApJ...159..379R Page 379[244] 1970ApJ...159..379R Page 379[244] 1970ApJ...159..379R Page 379[245] https://arxiv.org/pdf/astro-ph/0603143.pdf[245] https://arxiv.org/pdf/astro-ph/0603143.pdf[245] https://arxiv.org/pdf/astro-ph/0603143.pdf[245] https://arxiv.org/pdf/astro-ph/0603143.pdf[246] https://www.cambridge.org/core/services/aop-cambridge-core/content/view/88D75CC31EBBA30CF2F5028EA31E3FED/S0074180900000309a.pdf/comparison_of_dynamical_models_of_the_andromeda_nebula_and_the_galaxy.pdf[246] https://www.cambridge.org/core/services/aop-cambridge-core/content/view/88D75CC31EBBA30CF2F5028EA31E3FED/S0074180900000309a.pdf/comparison_of_dynamical_models_of_the_andromeda_nebula_and_the_galaxy.pdf[246] https://www.cambridge.org/core/services/aop-cambridge-core/content/view/88D75CC31EBBA30CF2F5028EA31E3FED/S0074180900000309a.pdf/comparison_of_dynamical_models_of_the_andromeda_nebula_and_the_galaxy.pdf[246] https://www.cambridge.org/core/services/aop-cambridge-core/content/view/88D75CC31EBBA30CF2F5028EA31E3FED/S0074180900000309a.pdf/comparison_of_dynamical_models_of_the_andromeda_nebula_and_the_galaxy.pdf[247] 1973A&A....26..483R Page 483[247] 1973A&A....26..483R Page 483[247] 1973A&A....26..483R Page 483[247] 1973A&A....26..483R Page 483[248] 1975ApJ...200..439B Page 439[248] 1975ApJ...200..439B Page 439[248] 1975ApJ...200..439B Page 439[248] 1975ApJ...200..439B Page 439[249] 1983MNRAS.203..735C Page 735[249] 1983MNRAS.203..735C Page 735[249] 1983MNRAS.203..735C Page 735[249] 1983MNRAS.203..735C Page 735[250] 1991ApJ...368...60P Page 60[250] 1991ApJ...368...60P Page 60[250] 1991ApJ...368...60P Page 60[250] 1991ApJ...368...60P Page 60[251] ShieldSquare Captcha[251] ShieldSquare Captcha[251] ShieldSquare Captcha[251] ShieldSquare Captcha[252] ShieldSquare Captcha[252] ShieldSquare Captcha[252] ShieldSquare Captcha[252] ShieldSquare Captcha[253] https://iopscience.iop.org/article/10.1088/0004-637X/718/1/60/pdf[253] https://iopscience.iop.org/article/10.1088/0004-637X/718/1/60/pdf[253] https://iopscience.iop.org/article/10.1088/0004-637X/718/1/60/pdf[253] https://iopscience.iop.org/article/10.1088/0004-637X/718/1/60/pdf[254] rise and fall of a challenger: the Bullet Cluster in Λ cold dark matter simulations[254] rise and fall of a challenger: the Bullet Cluster in Λ cold dark matter simulations[254] rise and fall of a challenger: the Bullet Cluster in Λ cold dark matter simulations[254] rise and fall of a challenger: the Bullet Cluster in Λ cold dark matter simulations[255] The Behavior of Hadron Collisions at Extreme Energies[255] The Behavior of Hadron Collisions at Extreme Energies[255] The Behavior of Hadron Collisions at Extreme Energies[255] The Behavior of Hadron Collisions at Extreme Energies[256] https://journals.aps.org/prl/pdf/10.1103/PhysRevLett.23.930[256] https://journals.aps.org/prl/pdf/10.1103/PhysRevLett.23.930[256] https://journals.aps.org/prl/pdf/10.1103/PhysRevLett.23.930[256] https://journals.aps.org/prl/pdf/10.1103/PhysRevLett.23.930[257] Observed Behavior of Highly Inelastic Electron-Proton Scattering[257] Observed Behavior of Highly Inelastic Electron-Proton Scattering[257] Observed Behavior of Highly Inelastic Electron-Proton Scattering[257] Observed Behavior of Highly Inelastic Electron-Proton Scattering[258] https://journals.aps.org/pr/pdf/10.1103/PhysRev.96.191[258] https://journals.aps.org/pr/pdf/10.1103/PhysRev.96.191[258] https://journals.aps.org/pr/pdf/10.1103/PhysRev.96.191[258] https://journals.aps.org/pr/pdf/10.1103/PhysRev.96.191[259] https://citeseerx.ist.psu.edu/viewdoc/download;jsessionid=ACF35E7F028D7EA8D4654DD5C565A570?doi=10.1.1.453.4712&rep=rep1&type=pdf[259] https://citeseerx.ist.psu.edu/viewdoc/download;jsessionid=ACF35E7F028D7EA8D4654DD5C565A570?doi=10.1.1.453.4712&rep=rep1&type=pdf[259] https://citeseerx.ist.psu.edu/viewdoc/download;jsessionid=ACF35E7F028D7EA8D4654DD5C565A570?doi=10.1.1.453.4712&rep=rep1&type=pdf[259] https://citeseerx.ist.psu.edu/viewdoc/download;jsessionid=ACF35E7F028D7EA8D4654DD5C565A570?doi=10.1.1.453.4712&rep=rep1&type=pdf[260] https://journals.aps.org/prl/pdf/10.1103/PhysRevLett.30.1343[260] https://journals.aps.org/prl/pdf/10.1103/PhysRevLett.30.1343[260] https://journals.aps.org/prl/pdf/10.1103/PhysRevLett.30.1343[260] https://journals.aps.org/prl/pdf/10.1103/PhysRevLett.30.1343[261] https://journals.aps.org/prl/pdf/10.1103/PhysRevLett.30.1346[261] https://journals.aps.org/prl/pdf/10.1103/PhysRevLett.30.1346[261] https://journals.aps.org/prl/pdf/10.1103/PhysRevLett.30.1346[261] https://journals.aps.org/prl/pdf/10.1103/PhysRevLett.30.1346[262] Confinement of quarks[262] Confinement of quarks[262] Confinement of quarks[262] Confinement of quarks[263] High-Precision Lattice QCD Confronts Experiment[263] High-Precision Lattice QCD Confronts Experiment[263] High-Precision Lattice QCD Confronts Experiment[263] High-Precision Lattice QCD Confronts Experiment[264] https://arxiv.org/pdf/hep-lat/0304004.pdf[264] https://arxiv.org/pdf/hep-lat/0304004.pdf[264] https://arxiv.org/pdf/hep-lat/0304004.pdf[264] https://arxiv.org/pdf/hep-lat/0304004.pdf[265] http://www.claymath.org/sites/default/files/yangmills.pdf[265] http://www.claymath.org/sites/default/files/yangmills.pdf[265] http://www.claymath.org/sites/default/files/yangmills.pdf[265] http://www.claymath.org/sites/default/files/yangmills.pdf[266] Black hole explosions?[266] Black hole explosions?[266] Black hole explosions?[266] Black hole explosions?[267] Particle creation by black holes[267] Particle creation by black holes[267] Particle creation by black holes[267] Particle creation by black holes[268] Particle emission rates from a black hole: Massless particles from an uncharged, nonrotating hole[268] Particle emission rates from a black hole: Massless particles from an uncharged, nonrotating hole[268] Particle emission rates from a black hole: Massless particles from an uncharged, nonrotating hole[268] Particle emission rates from a black hole: Massless particles from an uncharged, nonrotating hole[269] https://arxiv.org/pdf/0709.2380.pdf[269] https://arxiv.org/pdf/0709.2380.pdf[269] https://arxiv.org/pdf/0709.2380.pdf[269] https://arxiv.org/pdf/0709.2380.pdf[270] http://old.phys.huji.ac.il/~bekenste/PRD23-287-1981.pdf[270] http://old.phys.huji.ac.il/~bekenste/PRD23-287-1981.pdf[270] http://old.phys.huji.ac.il/~bekenste/PRD23-287-1981.pdf[270] http://old.phys.huji.ac.il/~bekenste/PRD23-287-1981.pdf[271] https://arxiv.org/pdf/hep-th/9209058.pdf[271] https://arxiv.org/pdf/hep-th/9209058.pdf[271] https://arxiv.org/pdf/hep-th/9209058.pdf[271] https://arxiv.org/pdf/hep-th/9209058.pdf[272] Soft Hair on Black Holes[272] Soft Hair on Black Holes[272] Soft Hair on Black Holes[272] Soft Hair on Black Holes[273] https://arxiv.org/pdf/1601.00921.pdf[273] https://arxiv.org/pdf/1601.00921.pdf[273] https://arxiv.org/pdf/1601.00921.pdf[273] https://arxiv.org/pdf/1601.00921.pdf[274] Black Holes Have Soft Quantum Hair[274] Black Holes Have Soft Quantum Hair[274] Black Holes Have Soft Quantum Hair[274] Black Holes Have Soft Quantum Hair[275] On the quantum structure of a black hole[275] On the quantum structure of a black hole[275] On the quantum structure of a black hole[275] On the quantum structure of a black hole[276] Black-hole evaporation and ultrashort distances[276] Black-hole evaporation and ultrashort distances[276] Black-hole evaporation and ultrashort distances[276] Black-hole evaporation and ultrashort distances[277] Hawking radiation without trans-Planckian frequencies[277] Hawking radiation without trans-Planckian frequencies[277] Hawking radiation without trans-Planckian frequencies[277] Hawking radiation without trans-Planckian frequencies[278] https://arxiv.org/pdf/hep-th/9506121.pdf[278] https://arxiv.org/pdf/hep-th/9506121.pdf[278] https://arxiv.org/pdf/hep-th/9506121.pdf[278] https://arxiv.org/pdf/hep-th/9506121.pdf[279] Information in black hole radiation[279] Information in black hole radiation[279] Information in black hole radiation[279] Information in black hole radiation[280] https://arxiv.org/pdf/hep-th/9306083.pdf[280] https://arxiv.org/pdf/hep-th/9306083.pdf[280] https://arxiv.org/pdf/hep-th/9306083.pdf[280] https://arxiv.org/pdf/hep-th/9306083.pdf[281] Average entropy of a subsystem[281] Average entropy of a subsystem[281] Average entropy of a subsystem[281] Average entropy of a subsystem[282] https://arxiv.org/pdf/gr-qc/9305007.pdf[282] https://arxiv.org/pdf/gr-qc/9305007.pdf[282] https://arxiv.org/pdf/gr-qc/9305007.pdf[282] https://arxiv.org/pdf/gr-qc/9305007.pdf[283] Black holes: complementarity or firewalls?[283] Black holes: complementarity or firewalls?[283] Black holes: complementarity or firewalls?[283] Black holes: complementarity or firewalls?[284] https://arxiv.org/pdf/1207.3123.pdf[284] https://arxiv.org/pdf/1207.3123.pdf[284] https://arxiv.org/pdf/1207.3123.pdf[284] https://arxiv.org/pdf/1207.3123.pdf[285] https://arxiv.org/pdf/1304.6483.pdf[285] https://arxiv.org/pdf/1304.6483.pdf[285] https://arxiv.org/pdf/1304.6483.pdf[285] https://arxiv.org/pdf/1304.6483.pdf[286] https://arxiv.org/pdf/1307.4706.pdf[286] https://arxiv.org/pdf/1307.4706.pdf[286] https://arxiv.org/pdf/1307.4706.pdf[286] https://arxiv.org/pdf/1307.4706.pdf[287] Astrophysics: Fire in the hole![287] Astrophysics: Fire in the hole![287] Astrophysics: Fire in the hole![287] Astrophysics: Fire in the hole![288] Theory of Hall Effect in a Two-Dimensional Electron System[288] Theory of Hall Effect in a Two-Dimensional Electron System[288] Theory of Hall Effect in a Two-Dimensional Electron System[288] Theory of Hall Effect in a Two-Dimensional Electron System[289] https://journals.aps.org/prl/pdf/10.1103/PhysRevLett.45.494[289] https://journals.aps.org/prl/pdf/10.1103/PhysRevLett.45.494[289] https://journals.aps.org/prl/pdf/10.1103/PhysRevLett.45.494[289] https://journals.aps.org/prl/pdf/10.1103/PhysRevLett.45.494[290] Quantized Hall conductivity in two dimensions[290] Quantized Hall conductivity in two dimensions[290] Quantized Hall conductivity in two dimensions[290] Quantized Hall conductivity in two dimensions[291] Quantization of particle transport[291] Quantization of particle transport[291] Quantization of particle transport[291] Quantization of particle transport[292] Quasiadiabatic continuation of quantum states: The stability of topological ground-state degeneracy and emergent gauge invariance[292] Quasiadiabatic continuation of quantum states: The stability of topological ground-state degeneracy and emergent gauge invariance[292] Quasiadiabatic continuation of quantum states: The stability of topological ground-state degeneracy and emergent gauge invariance[292] Quasiadiabatic continuation of quantum states: The stability of topological ground-state degeneracy and emergent gauge invariance[293] https://arxiv.org/pdf/cond-mat/0503554.pdf[293] https://arxiv.org/pdf/cond-mat/0503554.pdf[293] https://arxiv.org/pdf/cond-mat/0503554.pdf[293] https://arxiv.org/pdf/cond-mat/0503554.pdf[294] https://arxiv.org/pdf/1001.5280.pdf[294] https://arxiv.org/pdf/1001.5280.pdf[294] https://arxiv.org/pdf/1001.5280.pdf[294] https://arxiv.org/pdf/1001.5280.pdf[295] Quantization of Hall Conductance for Interacting Electrons on a Torus[295] Quantization of Hall Conductance for Interacting Electrons on a Torus[295] Quantization of Hall Conductance for Interacting Electrons on a Torus[295] Quantization of Hall Conductance for Interacting Electrons on a Torus[296] https://arxiv.org/pdf/0911.4706.pdf[296] https://arxiv.org/pdf/0911.4706.pdf[296] https://arxiv.org/pdf/0911.4706.pdf[296] https://arxiv.org/pdf/0911.4706.pdf[297] https://www.scientificamerican.com/article/how-scientists-solved-one-of-the-greatest-open-questions-in-quantum-physics/[297] https://www.scientificamerican.com/article/how-scientists-solved-one-of-the-greatest-open-questions-in-quantum-physics/[297] https://www.scientificamerican.com/article/how-scientists-solved-one-of-the-greatest-open-questions-in-quantum-physics/[297] https://www.scientificamerican.com/article/how-scientists-solved-one-of-the-greatest-open-questions-in-quantum-physics/[298] Resonant Tunneling in the Quantum Hall Regime: Measurement of Fractional Charge[298] Resonant Tunneling in the Quantum Hall Regime: Measurement of Fractional Charge[298] Resonant Tunneling in the Quantum Hall Regime: Measurement of Fractional Charge[298] Resonant Tunneling in the Quantum Hall Regime: Measurement of Fractional Charge[299] Observation of the $\mathit{e}\mathit{/}3$ Fractionally Charged Laughlin Quasiparticle[299] Observation of the $\mathit{e}\mathit{/}3$ Fractionally Charged Laughlin Quasiparticle[299] Observation of the $\mathit{e}\mathit{/}3$ Fractionally Charged Laughlin Quasiparticle[299] Observation of the $\mathit{e}\mathit{/}3$ Fractionally Charged Laughlin Quasiparticle[300] https://arxiv.org/pdf/cond-mat/9706307.pdf[300] https://arxiv.org/pdf/cond-mat/9706307.pdf[300] https://arxiv.org/pdf/cond-mat/9706307.pdf[300] https://arxiv.org/pdf/cond-mat/9706307.pdf[301] Quantum Spin Hall Effect in Graphene[301] Quantum Spin Hall Effect in Graphene[301] Quantum Spin Hall Effect in Graphene[301] Quantum Spin Hall Effect in Graphene[302] https://arxiv.org/pdf/cond-mat/0411737.pdf[302] https://arxiv.org/pdf/cond-mat/0411737.pdf[302] https://arxiv.org/pdf/cond-mat/0411737.pdf[302] https://arxiv.org/pdf/cond-mat/0411737.pdf[303] https://journals.aps.org/prl/pdf/10.1103/PhysRevLett.61.2015[303] https://journals.aps.org/prl/pdf/10.1103/PhysRevLett.61.2015[303] https://journals.aps.org/prl/pdf/10.1103/PhysRevLett.61.2015[303] https://journals.aps.org/prl/pdf/10.1103/PhysRevLett.61.2015[304] https://journals.aps.org/prd/pdf/10.1103/PhysRevD.23.347[304] https://journals.aps.org/prd/pdf/10.1103/PhysRevD.23.347[304] https://journals.aps.org/prd/pdf/10.1103/PhysRevD.23.347[304] https://journals.aps.org/prd/pdf/10.1103/PhysRevD.23.347[305] https://www.researchgate.net/profile/Alexei_Starobinsky/publication/222625284_A_New_Type_of_Isotropic_Cosmological_Models_Without_Singularity/links/59dc81e6a6fdcc1ec8a6fa4b/A-New-Type-of-Isotropic-Cosmological-Models-Without-Singularity.pdf[305] https://www.researchgate.net/profile/Alexei_Starobinsky/publication/222625284_A_New_Type_of_Isotropic_Cosmological_Models_Without_Singularity/links/59dc81e6a6fdcc1ec8a6fa4b/A-New-Type-of-Isotropic-Cosmological-Models-Without-Singularity.pdf[305] https://www.researchgate.net/profile/Alexei_Starobinsky/publication/222625284_A_New_Type_of_Isotropic_Cosmological_Models_Without_Singularity/links/59dc81e6a6fdcc1ec8a6fa4b/A-New-Type-of-Isotropic-Cosmological-Models-Without-Singularity.pdf[305] https://www.researchgate.net/profile/Alexei_Starobinsky/publication/222625284_A_New_Type_of_Isotropic_Cosmological_Models_Without_Singularity/links/59dc81e6a6fdcc1ec8a6fa4b/A-New-Type-of-Isotropic-Cosmological-Models-Without-Singularity.pdf[306] http://articles.adsabs.harvard.edu/pdf/1980ApJ...241L..59K[306] http://articles.adsabs.harvard.edu/pdf/1980ApJ...241L..59K[306] http://articles.adsabs.harvard.edu/pdf/1980ApJ...241L..59K[306] http://articles.adsabs.harvard.edu/pdf/1980ApJ...241L..59K[307] Cosmological baryon-number domain structure and the first order phase transition of a vacuum[307] Cosmological baryon-number domain structure and the first order phase transition of a vacuum[307] Cosmological baryon-number domain structure and the first order phase transition of a vacuum[307] Cosmological baryon-number domain structure and the first order phase transition of a vacuum[308] Monopole production in the very early universe in a first-order phase transition[308] Monopole production in the very early universe in a first-order phase transition[308] Monopole production in the very early universe in a first-order phase transition[308] Monopole production in the very early universe in a first-order phase transition[309] A new inflationary universe scenario: A possible solution of the horizon, flatness, homogeneity, isotropy and primordial monopole problems[309] A new inflationary universe scenario: A possible solution of the horizon, flatness, homogeneity, isotropy and primordial monopole problems[309] A new inflationary universe scenario: A possible solution of the horizon, flatness, homogeneity, isotropy and primordial monopole problems[309] A new inflationary universe scenario: A possible solution of the horizon, flatness, homogeneity, isotropy and primordial monopole problems[310] Cosmology for Grand Unified Theories with Radiatively Induced Symmetry Breaking[310] Cosmology for Grand Unified Theories with Radiatively Induced Symmetry Breaking[310] Cosmology for Grand Unified Theories with Radiatively Induced Symmetry Breaking[310] Cosmology for Grand Unified Theories with Radiatively Induced Symmetry Breaking[311] http://www.jetpletters.ac.ru/ps/1510/article_23079.pdf[311] http://www.jetpletters.ac.ru/ps/1510/article_23079.pdf[311] http://www.jetpletters.ac.ru/ps/1510/article_23079.pdf[311] http://www.jetpletters.ac.ru/ps/1510/article_23079.pdf[312] http://jetp.ac.ru/cgi-bin/dn/e_056_02_0258.pdf[312] http://jetp.ac.ru/cgi-bin/dn/e_056_02_0258.pdf[312] http://jetp.ac.ru/cgi-bin/dn/e_056_02_0258.pdf[312] http://jetp.ac.ru/cgi-bin/dn/e_056_02_0258.pdf[313] The development of irregularities in a single bubble inflationary universe[313] The development of irregularities in a single bubble inflationary universe[313] The development of irregularities in a single bubble inflationary universe[313] The development of irregularities in a single bubble inflationary universe[314] https://reader.elsevier.com/reader/sd/pii/037026938290541X?token=51C79D8D8B4BE8E243F2AFBBDFB31C8EEE6FA8BAC4FE9A51A9C0B666E14668FAF0C3A9C95E840D62311D90B08DA8BC69[314] https://reader.elsevier.com/reader/sd/pii/037026938290541X?token=51C79D8D8B4BE8E243F2AFBBDFB31C8EEE6FA8BAC4FE9A51A9C0B666E14668FAF0C3A9C95E840D62311D90B08DA8BC69[314] https://reader.elsevier.com/reader/sd/pii/037026938290541X?token=51C79D8D8B4BE8E243F2AFBBDFB31C8EEE6FA8BAC4FE9A51A9C0B666E14668FAF0C3A9C95E840D62311D90B08DA8BC69[314] https://reader.elsevier.com/reader/sd/pii/037026938290541X?token=51C79D8D8B4BE8E243F2AFBBDFB31C8EEE6FA8BAC4FE9A51A9C0B666E14668FAF0C3A9C95E840D62311D90B08DA8BC69[315] Fluctuations in the New Inflationary Universe[315] Fluctuations in the New Inflationary Universe[315] Fluctuations in the New Inflationary Universe[315] Fluctuations in the New Inflationary Universe[316] Spontaneous creation of almost scale-free density perturbations in an inflationary universe[316] Spontaneous creation of almost scale-free density perturbations in an inflationary universe[316] Spontaneous creation of almost scale-free density perturbations in an inflationary universe[316] Spontaneous creation of almost scale-free density perturbations in an inflationary universe[317] Inflationary Predictions for Scalar and Tensor Fluctuations Reconsidered[317] Inflationary Predictions for Scalar and Tensor Fluctuations Reconsidered[317] Inflationary Predictions for Scalar and Tensor Fluctuations Reconsidered[317] Inflationary Predictions for Scalar and Tensor Fluctuations Reconsidered[318] https://arxiv.org/pdf/astro-ph/0507455[318] https://arxiv.org/pdf/astro-ph/0507455[318] https://arxiv.org/pdf/astro-ph/0507455[318] https://arxiv.org/pdf/astro-ph/0507455[319] Cosmological constraints from the SDSS luminous red galaxies[319] Cosmological constraints from the SDSS luminous red galaxies[319] Cosmological constraints from the SDSS luminous red galaxies[319] Cosmological constraints from the SDSS luminous red galaxies[320] https://arxiv.org/pdf/astro-ph/0608632.pdf[320] https://arxiv.org/pdf/astro-ph/0608632.pdf[320] https://arxiv.org/pdf/astro-ph/0608632.pdf[320] https://arxiv.org/pdf/astro-ph/0608632.pdf[321] https://www.worldscientific.com/doi/pdf/10.1142/S0217732304014252[321] https://www.worldscientific.com/doi/pdf/10.1142/S0217732304014252[321] https://www.worldscientific.com/doi/pdf/10.1142/S0217732304014252[321] https://www.worldscientific.com/doi/pdf/10.1142/S0217732304014252[322] What does inflation really predict?[322] What does inflation really predict?[322] What does inflation really predict?[322] What does inflation really predict?[323] https://arxiv.org/pdf/astro-ph/0410281.pdf[323] https://arxiv.org/pdf/astro-ph/0410281.pdf[323] https://arxiv.org/pdf/astro-ph/0410281.pdf[323] https://arxiv.org/pdf/astro-ph/0410281.pdf[324] https://www.aanda.org/articles/aa/full_html/2016/10/aa25830-15/aa25830-15.html[324] https://www.aanda.org/articles/aa/full_html/2016/10/aa25830-15/aa25830-15.html[324] https://www.aanda.org/articles/aa/full_html/2016/10/aa25830-15/aa25830-15.html[324] https://www.aanda.org/articles/aa/full_html/2016/10/aa25830-15/aa25830-15.html[325] https://www.aanda.org/articles/aa/full_html/2016/10/aa25898-15/aa25898-15.html[325] https://www.aanda.org/articles/aa/full_html/2016/10/aa25898-15/aa25898-15.html[325] https://www.aanda.org/articles/aa/full_html/2016/10/aa25898-15/aa25898-15.html[325] https://www.aanda.org/articles/aa/full_html/2016/10/aa25898-15/aa25898-15.html[326] https://iopscience.iop.org/article/10.1086/513700/pdf[326] https://iopscience.iop.org/article/10.1086/513700/pdf[326] https://iopscience.iop.org/article/10.1086/513700/pdf[326] https://iopscience.iop.org/article/10.1086/513700/pdf[327] https://journals.aps.org/prl/pdf/10.1103/PhysRevLett.112.241101[327] https://journals.aps.org/prl/pdf/10.1103/PhysRevLett.112.241101[327] https://journals.aps.org/prl/pdf/10.1103/PhysRevLett.112.241101[327] https://journals.aps.org/prl/pdf/10.1103/PhysRevLett.112.241101[328] https://www.aanda.org/articles/aa/abs/2016/02/aa25034-14/aa25034-14.html[328] https://www.aanda.org/articles/aa/abs/2016/02/aa25034-14/aa25034-14.html[328] https://www.aanda.org/articles/aa/abs/2016/02/aa25034-14/aa25034-14.html[328] https://www.aanda.org/articles/aa/abs/2016/02/aa25034-14/aa25034-14.html[329] https://arxiv.org/pdf/1409.5738.pdf[329] https://arxiv.org/pdf/1409.5738.pdf[329] https://arxiv.org/pdf/1409.5738.pdf[329] https://arxiv.org/pdf/1409.5738.pdf[330] Five years after BICEP2[330] Five years after BICEP2[330] Five years after BICEP2[330] Five years after BICEP2[331] https://arxiv.org/pdf/1304.2785.pdf[331] https://arxiv.org/pdf/1304.2785.pdf[331] https://arxiv.org/pdf/1304.2785.pdf[331] https://arxiv.org/pdf/1304.2785.pdf[332] https://arxiv.org/pdf/1402.6980.pdf[332] https://arxiv.org/pdf/1402.6980.pdf[332] https://arxiv.org/pdf/1402.6980.pdf[332] https://arxiv.org/pdf/1402.6980.pdf[333] https://arxiv.org/pdf/1512.09010.pdf[333] https://arxiv.org/pdf/1512.09010.pdf[333] https://arxiv.org/pdf/1512.09010.pdf[333] https://arxiv.org/pdf/1512.09010.pdf[334] Sailing through the big crunch-big bang transition[334] Sailing through the big crunch-big bang transition[334] Sailing through the big crunch-big bang transition[334] Sailing through the big crunch-big bang transition[335] https://www.scientificamerican.com/article/cosmic-inflation-theory-faces-challenges/[335] https://www.scientificamerican.com/article/cosmic-inflation-theory-faces-challenges/[335] https://www.scientificamerican.com/article/cosmic-inflation-theory-faces-challenges/[335] https://www.scientificamerican.com/article/cosmic-inflation-theory-faces-challenges/[336] https://arxiv.org/pdf/1103.2271.pdf[336] https://arxiv.org/pdf/1103.2271.pdf[336] https://arxiv.org/pdf/1103.2271.pdf[336] https://arxiv.org/pdf/1103.2271.pdf[337] Influence of Retardation on the London–van der Waals Forces[337] Influence of Retardation on the London–van der Waals Forces[337] Influence of Retardation on the London–van der Waals Forces[337] Influence of Retardation on the London–van der Waals Forces[338] The Influence of Retardation on the London-van der Waals Forces[338] The Influence of Retardation on the London-van der Waals Forces[338] The Influence of Retardation on the London-van der Waals Forces[338] The Influence of Retardation on the London-van der Waals Forces[339] https://www.mit.edu/~kardar/research/seminars/Casimir/PR-CasimirPolder48.pdf[339] https://www.mit.edu/~kardar/research/seminars/Casimir/PR-CasimirPolder48.pdf[339] https://www.mit.edu/~kardar/research/seminars/Casimir/PR-CasimirPolder48.pdf[339] https://www.mit.edu/~kardar/research/seminars/Casimir/PR-CasimirPolder48.pdf[340] https://www.dwc.knaw.nl/DL/publications/PU00018547.pdf[340] https://www.dwc.knaw.nl/DL/publications/PU00018547.pdf[340] https://www.dwc.knaw.nl/DL/publications/PU00018547.pdf[340] https://www.dwc.knaw.nl/DL/publications/PU00018547.pdf[341] Casimir effect and the quantum vacuum[341] Casimir effect and the quantum vacuum[341] Casimir effect and the quantum vacuum[341] Casimir effect and the quantum vacuum[342] https://arxiv.org/pdf/hep-th/0503158.pdf[342] https://arxiv.org/pdf/hep-th/0503158.pdf[342] https://arxiv.org/pdf/hep-th/0503158.pdf[342] https://arxiv.org/pdf/hep-th/0503158.pdf[343] Demonstration of the Casimir Force in the 0.6 to $6\ensuremath{\mu}m$ Range[343] Demonstration of the Casimir Force in the 0.6 to $6\ensuremath{\mu}m$ Range[343] Demonstration of the Casimir Force in the 0.6 to $6\ensuremath{\mu}m$ Range[343] Demonstration of the Casimir Force in the 0.6 to $6\ensuremath{\mu}m$ Range[344] Precision Measurement of the Casimir Force from 0.1 to $0.9\mathit{\ensuremath{\mu}}m$[344] Precision Measurement of the Casimir Force from 0.1 to $0.9\mathit{\ensuremath{\mu}}m$[344] Precision Measurement of the Casimir Force from 0.1 to $0.9\mathit{\ensuremath{\mu}}m$[344] Precision Measurement of the Casimir Force from 0.1 to $0.9\mathit{\ensuremath{\mu}}m$[345] https://arxiv.org/pdf/physics/9805038.pdf[345] https://arxiv.org/pdf/physics/9805038.pdf[345] https://arxiv.org/pdf/physics/9805038.pdf[345] https://arxiv.org/pdf/physics/9805038.pdf[346] Measurement of the Casimir Force between Parallel Metallic Surfaces[346] Measurement of the Casimir Force between Parallel Metallic Surfaces[346] Measurement of the Casimir Force between Parallel Metallic Surfaces[346] Measurement of the Casimir Force between Parallel Metallic Surfaces[347] https://arxiv.org/pdf/quant-ph/0203002.pdf[347] https://arxiv.org/pdf/quant-ph/0203002.pdf[347] https://arxiv.org/pdf/quant-ph/0203002.pdf[347] https://arxiv.org/pdf/quant-ph/0203002.pdf[348] https://www.ias.ac.in/article/fulltext/reso/009/05/0091-0095[348] https://www.ias.ac.in/article/fulltext/reso/009/05/0091-0095[348] https://www.ias.ac.in/article/fulltext/reso/009/05/0091-0095[348] https://www.ias.ac.in/article/fulltext/reso/009/05/0091-0095[349] Late stages of crunch[349] Late stages of crunch[349] Late stages of crunch[349] Late stages of crunch[350] The Big Crunch | MRS Bulletin | Cambridge Core[350] The Big Crunch | MRS Bulletin | Cambridge Core[350] The Big Crunch | MRS Bulletin | Cambridge Core[350] The Big Crunch | MRS Bulletin | Cambridge Core[351] https://arxiv.org/pdf/gr-qc/9704038.pdf[351] https://arxiv.org/pdf/gr-qc/9704038.pdf[351] https://arxiv.org/pdf/gr-qc/9704038.pdf[351] https://arxiv.org/pdf/gr-qc/9704038.pdf[352] The big crunch[352] The big crunch[352] The big crunch[352] The big crunch[353] https://iopscience.iop.org/article/10.1086/307221/pdf[353] https://iopscience.iop.org/article/10.1086/307221/pdf[353] https://iopscience.iop.org/article/10.1086/307221/pdf[353] https://iopscience.iop.org/article/10.1086/307221/pdf[354] 1968ApJ...152L.149S Page L149[354] 1968ApJ...152L.149S Page L149[354] 1968ApJ...152L.149S Page L149[354] 1968ApJ...152L.149S Page L149[355] 1985ApJ...294...81T Page 81[355] 1985ApJ...294...81T Page 81[355] 1985ApJ...294...81T Page 81[355] 1985ApJ...294...81T Page 81[356] 1991ApJ...379..466B Page 466[356] 1991ApJ...379..466B Page 466[356] 1991ApJ...379..466B Page 466[356] 1991ApJ...379..466B Page 466[357] 1994ApJ...420...33B Page 33[357] 1994ApJ...420...33B Page 33[357] 1994ApJ...420...33B Page 33[357] 1994ApJ...420...33B Page 33[358] The Case for a Hubble Constant of 30 km/s/Mpc[358] The Case for a Hubble Constant of 30 km/s/Mpc[358] The Case for a Hubble Constant of 30 km/s/Mpc[358] The Case for a Hubble Constant of 30 km/s/Mpc[359] https://iopscience.iop.org/article/10.1086/304583/pdf[359] https://iopscience.iop.org/article/10.1086/304583/pdf[359] https://iopscience.iop.org/article/10.1086/304583/pdf[359] https://iopscience.iop.org/article/10.1086/304583/pdf[360] 1987ApJ...317....1D Page 1[360] 1987ApJ...317....1D Page 1[360] 1987ApJ...317....1D Page 1[360] 1987ApJ...317....1D Page 1[361] 1992ApJ...392...35B Page 35[361] 1992ApJ...392...35B Page 35[361] 1992ApJ...392...35B Page 35[361] 1992ApJ...392...35B Page 35[362] 1995ApJ...449..413M Page 413[362] 1995ApJ...449..413M Page 413[362] 1995ApJ...449..413M Page 413[362] 1995ApJ...449..413M Page 413[363] https://arxiv.org/pdf/astro-ph/9512156.pdf[363] https://arxiv.org/pdf/astro-ph/9512156.pdf[363] https://arxiv.org/pdf/astro-ph/9512156.pdf[363] https://arxiv.org/pdf/astro-ph/9512156.pdf[364] ShieldSquare Captcha[364] ShieldSquare Captcha[364] ShieldSquare Captcha[364] ShieldSquare Captcha[365] https://iopscience.iop.org/article/10.1086/303576/pdf[365] https://iopscience.iop.org/article/10.1086/303576/pdf[365] https://iopscience.iop.org/article/10.1086/303576/pdf[365] https://iopscience.iop.org/article/10.1086/303576/pdf[366] https://iopscience.iop.org/article/10.1086/306308/pdf[366] https://iopscience.iop.org/article/10.1086/306308/pdf[366] https://iopscience.iop.org/article/10.1086/306308/pdf[366] https://iopscience.iop.org/article/10.1086/306308/pdf[367] https://iopscience.iop.org/article/10.1086/307004/pdf[367] https://iopscience.iop.org/article/10.1086/307004/pdf[367] https://iopscience.iop.org/article/10.1086/307004/pdf[367] https://iopscience.iop.org/article/10.1086/307004/pdf[368] Cepheid distance to M96 and the Hubble constant[368] Cepheid distance to M96 and the Hubble constant[368] Cepheid distance to M96 and the Hubble constant[368] Cepheid distance to M96 and the Hubble constant[369] ShieldSquare Captcha[369] ShieldSquare Captcha[369] ShieldSquare Captcha[369] ShieldSquare Captcha[370] ShieldSquare Captcha[370] ShieldSquare Captcha[370] ShieldSquare Captcha[370] ShieldSquare Captcha[371] ShieldSquare Captcha[371] ShieldSquare Captcha[371] ShieldSquare Captcha[371] ShieldSquare Captcha[372] ShieldSquare Captcha[372] ShieldSquare Captcha[372] ShieldSquare Captcha[372] ShieldSquare Captcha[373] ShieldSquare Captcha[373] ShieldSquare Captcha[373] ShieldSquare Captcha[373] ShieldSquare Captcha[374] ShieldSquare Captcha[374] ShieldSquare Captcha[374] ShieldSquare Captcha[374] ShieldSquare Captcha[375] ShieldSquare Captcha[375] ShieldSquare Captcha[375] ShieldSquare Captcha[375] ShieldSquare Captcha[376] ShieldSquare Captcha[376] ShieldSquare Captcha[376] ShieldSquare Captcha[376] ShieldSquare Captcha[377] https://iopscience.iop.org/article/10.1086/319449/fulltext/52239.text.html[377] https://iopscience.iop.org/article/10.1086/319449/fulltext/52239.text.html[377] https://iopscience.iop.org/article/10.1086/319449/fulltext/52239.text.html[377] https://iopscience.iop.org/article/10.1086/319449/fulltext/52239.text.html[378] https://www.aanda.org/articles/aa/pdf/2001/43/aa1494.pdf[378] https://www.aanda.org/articles/aa/pdf/2001/43/aa1494.pdf[378] https://www.aanda.org/articles/aa/pdf/2001/43/aa1494.pdf[378] https://www.aanda.org/articles/aa/pdf/2001/43/aa1494.pdf[379] ShieldSquare Captcha[379] ShieldSquare Captcha[379] ShieldSquare Captcha[379] ShieldSquare Captcha[380] Evidence for a non-zero Λ and a low matter density from a combined analysis of the 2dF Galaxy Redshift Survey and cosmic microwave background anisotropies[380] Evidence for a non-zero Λ and a low matter density from a combined analysis of the 2dF Galaxy Redshift Survey and cosmic microwave background anisotropies[380] Evidence for a non-zero Λ and a low matter density from a combined analysis of the 2dF Galaxy Redshift Survey and cosmic microwave background anisotropies[380] Evidence for a non-zero Λ and a low matter density from a combined analysis of the 2dF Galaxy Redshift Survey and cosmic microwave background anisotropies[381] https://iopscience.iop.org/article/10.1088/0004-637X/779/1/86/pdf[381] https://iopscience.iop.org/article/10.1088/0004-637X/779/1/86/pdf[381] https://iopscience.iop.org/article/10.1088/0004-637X/779/1/86/pdf[381] https://iopscience.iop.org/article/10.1088/0004-637X/779/1/86/pdf[382] https://arxiv.org/pdf/astro-ph/9909076.pdf[382] https://arxiv.org/pdf/astro-ph/9909076.pdf[382] https://arxiv.org/pdf/astro-ph/9909076.pdf[382] https://arxiv.org/pdf/astro-ph/9909076.pdf[383] https://arxiv.org/pdf/astro-ph/0408338.pdf[383] https://arxiv.org/pdf/astro-ph/0408338.pdf[383] https://arxiv.org/pdf/astro-ph/0408338.pdf[383] https://arxiv.org/pdf/astro-ph/0408338.pdf[384] The Hubble Constant[384] The Hubble Constant[384] The Hubble Constant[384] The Hubble Constant[385] 2013IAUS..289..255B Page 255[385] 2013IAUS..289..255B Page 255[385] 2013IAUS..289..255B Page 255[385] 2013IAUS..289..255B Page 255[386] https://www.aanda.org/articles/aa/abs/2014/11/aa21591-13/aa21591-13.html[386] https://www.aanda.org/articles/aa/abs/2014/11/aa21591-13/aa21591-13.html[386] https://www.aanda.org/articles/aa/abs/2014/11/aa21591-13/aa21591-13.html[386] https://www.aanda.org/articles/aa/abs/2014/11/aa21591-13/aa21591-13.html[387] ShieldSquare Captcha[387] ShieldSquare Captcha[387] ShieldSquare Captcha[387] ShieldSquare Captcha[388] https://www.scientificamerican.com/article/how-a-dispute-over-a-single-number-became-a-cosmological-crisis/[388] https://www.scientificamerican.com/article/how-a-dispute-over-a-single-number-became-a-cosmological-crisis/[388] https://www.scientificamerican.com/article/how-a-dispute-over-a-single-number-became-a-cosmological-crisis/[388] https://www.scientificamerican.com/article/how-a-dispute-over-a-single-number-became-a-cosmological-crisis/[389] ShieldSquare Captcha[389] ShieldSquare Captcha[389] ShieldSquare Captcha[389] ShieldSquare Captcha[390] ShieldSquare Captcha[390] ShieldSquare Captcha[390] ShieldSquare Captcha[390] ShieldSquare Captcha[391] https://www.aanda.org/articles/aa/pdf/forth/aa33910-18.pdf[391] https://www.aanda.org/articles/aa/pdf/forth/aa33910-18.pdf[391] https://www.aanda.org/articles/aa/pdf/forth/aa33910-18.pdf[391] https://www.aanda.org/articles/aa/pdf/forth/aa33910-18.pdf[392] ShieldSquare Captcha[392] ShieldSquare Captcha[392] ShieldSquare Captcha[392] ShieldSquare Captcha[393] ShieldSquare Captcha[393] ShieldSquare Captcha[393] ShieldSquare Captcha[393] ShieldSquare Captcha[394] ShieldSquare Captcha[394] ShieldSquare Captcha[394] ShieldSquare Captcha[394] ShieldSquare Captcha[395] https://phy-act1.princeton.edu/public/saiola/act_dr4_A20.pdf[395] https://phy-act1.princeton.edu/public/saiola/act_dr4_A20.pdf[395] https://phy-act1.princeton.edu/public/saiola/act_dr4_A20.pdf[395] https://phy-act1.princeton.edu/public/saiola/act_dr4_A20.pdf[396] https://phy-act1.princeton.edu/public/saiola/act_dr4_C20.pdf[396] https://phy-act1.princeton.edu/public/saiola/act_dr4_C20.pdf[396] https://phy-act1.princeton.edu/public/saiola/act_dr4_C20.pdf[396] https://phy-act1.princeton.edu/public/saiola/act_dr4_C20.pdf[397] Measuring the Hubble constant with a sample of kilonovae[397] Measuring the Hubble constant with a sample of kilonovae[397] Measuring the Hubble constant with a sample of kilonovae[397] Measuring the Hubble constant with a sample of kilonovae[398] Preposterous Universe[398] Preposterous Universe[398] Preposterous Universe[398] Preposterous Universe[399] Vacuum stability[399] Vacuum stability[399] Vacuum stability[399] Vacuum stability[400] A phantom menace? Cosmological consequences of a dark energy component with super-negative equation of state[400] A phantom menace? Cosmological consequences of a dark energy component with super-negative equation of state[400] A phantom menace? Cosmological consequences of a dark energy component with super-negative equation of state[400] A phantom menace? Cosmological consequences of a dark energy component with super-negative equation of state[401] https://arxiv.org/pdf/astro-ph/9908168.pdf[401] https://arxiv.org/pdf/astro-ph/9908168.pdf[401] https://arxiv.org/pdf/astro-ph/9908168.pdf[401] https://arxiv.org/pdf/astro-ph/9908168.pdf[402] Phantom Energy: Dark Energy with $w<\ensuremath{-}1$ Causes a Cosmic Doomsday[402] Phantom Energy: Dark Energy with $w<\ensuremath{-}1$ Causes a Cosmic Doomsday[402] Phantom Energy: Dark Energy with $w<\ensuremath{-}1$ Causes a Cosmic Doomsday[402] Phantom Energy: Dark Energy with $w<\ensuremath{-}1$ Causes a Cosmic Doomsday[403] The End of Everything[403] The End of Everything[403] The End of Everything[403] The End of Everything[404] Mass of the Higgs Boson[404] Mass of the Higgs Boson[404] Mass of the Higgs Boson[404] Mass of the Higgs Boson[405] https://cds.cern.ch/record/874049/files/CM-P00061607.pdf[405] https://cds.cern.ch/record/874049/files/CM-P00061607.pdf[405] https://cds.cern.ch/record/874049/files/CM-P00061607.pdf[405] https://cds.cern.ch/record/874049/files/CM-P00061607.pdf[406] https://arxiv.org/pdf/hep-ph/9504378.pdf[406] https://arxiv.org/pdf/hep-ph/9504378.pdf[406] https://arxiv.org/pdf/hep-ph/9504378.pdf[406] https://arxiv.org/pdf/hep-ph/9504378.pdf[407] https://arxiv.org/pdf/hep-ph/0009158.pdf[407] https://arxiv.org/pdf/hep-ph/0009158.pdf[407] https://arxiv.org/pdf/hep-ph/0009158.pdf[407] https://arxiv.org/pdf/hep-ph/0009158.pdf[408] https://arxiv.org/pdf/hep-ph/0208209.pdf[408] https://arxiv.org/pdf/hep-ph/0208209.pdf[408] https://arxiv.org/pdf/hep-ph/0208209.pdf[408] https://arxiv.org/pdf/hep-ph/0208209.pdf[409] Search for the Standard Model Higgs boson at LEP[409] Search for the Standard Model Higgs boson at LEP[409] Search for the Standard Model Higgs boson at LEP[409] Search for the Standard Model Higgs boson at LEP[410] https://iopscience.iop.org/article/10.1088/0954-3899/33/1/001/pdf[410] https://iopscience.iop.org/article/10.1088/0954-3899/33/1/001/pdf[410] https://iopscience.iop.org/article/10.1088/0954-3899/33/1/001/pdf[410] https://iopscience.iop.org/article/10.1088/0954-3899/33/1/001/pdf[411] Observation of Top Quark Production in $\overline{\mathit{p}}\mathit{p}$ Collisions with the Collider Detector at Fermilab[411] Observation of Top Quark Production in $\overline{\mathit{p}}\mathit{p}$ Collisions with the Collider Detector at Fermilab[411] Observation of Top Quark Production in $\overline{\mathit{p}}\mathit{p}$ Collisions with the Collider Detector at Fermilab[411] Observation of Top Quark Production in $\overline{\mathit{p}}\mathit{p}$ Collisions with the Collider Detector at Fermilab[412] https://arxiv.org/pdf/hep-ex/9503002.pdf[412] https://arxiv.org/pdf/hep-ex/9503002.pdf[412] https://arxiv.org/pdf/hep-ex/9503002.pdf[412] https://arxiv.org/pdf/hep-ex/9503002.pdf[413] Observation of the Top Quark[413] Observation of the Top Quark[413] Observation of the Top Quark[413] Observation of the Top Quark[414] https://arxiv.org/pdf/hep-ex/9503003.pdf[414] https://arxiv.org/pdf/hep-ex/9503003.pdf[414] https://arxiv.org/pdf/hep-ex/9503003.pdf[414] https://arxiv.org/pdf/hep-ex/9503003.pdf[415] https://arxiv.org/pdf/1207.0449.pdf[415] https://arxiv.org/pdf/1207.0449.pdf[415] https://arxiv.org/pdf/1207.0449.pdf[415] https://arxiv.org/pdf/1207.0449.pdf[416] https://journals.aps.org/prd/pdf/10.1103/PhysRevD.86.072004[416] https://journals.aps.org/prd/pdf/10.1103/PhysRevD.86.072004[416] https://journals.aps.org/prd/pdf/10.1103/PhysRevD.86.072004[416] https://journals.aps.org/prd/pdf/10.1103/PhysRevD.86.072004[417] https://journals.aps.org/prd/pdf/10.1103/PhysRevD.86.072010[417] https://journals.aps.org/prd/pdf/10.1103/PhysRevD.86.072010[417] https://journals.aps.org/prd/pdf/10.1103/PhysRevD.86.072010[417] https://journals.aps.org/prd/pdf/10.1103/PhysRevD.86.072010[418] Combined Search for the Standard Model Higgs Boson Decaying to a $b\overline{b}$ Pair Using the Full CDF Data Set[418] Combined Search for the Standard Model Higgs Boson Decaying to a $b\overline{b}$ Pair Using the Full CDF Data Set[418] Combined Search for the Standard Model Higgs Boson Decaying to a $b\overline{b}$ Pair Using the Full CDF Data Set[418] Combined Search for the Standard Model Higgs Boson Decaying to a $b\overline{b}$ Pair Using the Full CDF Data Set[419] https://arxiv.org/pdf/1207.1707.pdf[419] https://arxiv.org/pdf/1207.1707.pdf[419] https://arxiv.org/pdf/1207.1707.pdf[419] https://arxiv.org/pdf/1207.1707.pdf[420] Combined Search for the Standard Model Higgs Boson Decaying to $b\overline{b}$ Using the D0 Run II Data Set[420] Combined Search for the Standard Model Higgs Boson Decaying to $b\overline{b}$ Using the D0 Run II Data Set[420] Combined Search for the Standard Model Higgs Boson Decaying to $b\overline{b}$ Using the D0 Run II Data Set[420] Combined Search for the Standard Model Higgs Boson Decaying to $b\overline{b}$ Using the D0 Run II Data Set[421] https://arxiv.org/pdf/1207.6631.pdf[421] https://arxiv.org/pdf/1207.6631.pdf[421] https://arxiv.org/pdf/1207.6631.pdf[421] https://arxiv.org/pdf/1207.6631.pdf[422] Evidence for a Particle Produced in Association with Weak Bosons and Decaying to a Bottom-Antibottom Quark Pair in Higgs Boson Searches at the Tevatron[422] Evidence for a Particle Produced in Association with Weak Bosons and Decaying to a Bottom-Antibottom Quark Pair in Higgs Boson Searches at the Tevatron[422] Evidence for a Particle Produced in Association with Weak Bosons and Decaying to a Bottom-Antibottom Quark Pair in Higgs Boson Searches at the Tevatron[422] Evidence for a Particle Produced in Association with Weak Bosons and Decaying to a Bottom-Antibottom Quark Pair in Higgs Boson Searches at the Tevatron[423] https://arxiv.org/pdf/1207.6436.pdf[423] https://arxiv.org/pdf/1207.6436.pdf[423] https://arxiv.org/pdf/1207.6436.pdf[423] https://arxiv.org/pdf/1207.6436.pdf[424] Observation of a new particle in the search for the Standard Model Higgs boson with the ATLAS detector at the LHC[424] Observation of a new particle in the search for the Standard Model Higgs boson with the ATLAS detector at the LHC[424] Observation of a new particle in the search for the Standard Model Higgs boson with the ATLAS detector at the LHC[424] Observation of a new particle in the search for the Standard Model Higgs boson with the ATLAS detector at the LHC[425] Observation of a new boson at a mass of 125 GeV with the CMS experiment at the LHC[425] Observation of a new boson at a mass of 125 GeV with the CMS experiment at the LHC[425] Observation of a new boson at a mass of 125 GeV with the CMS experiment at the LHC[425] Observation of a new boson at a mass of 125 GeV with the CMS experiment at the LHC[426] Constraining anomalous Higgs boson interactions[426] Constraining anomalous Higgs boson interactions[426] Constraining anomalous Higgs boson interactions[426] Constraining anomalous Higgs boson interactions[427] Higgs boson mass and new physics[427] Higgs boson mass and new physics[427] Higgs boson mass and new physics[427] Higgs boson mass and new physics[428] The Higgs-boson lineshape[428] The Higgs-boson lineshape[428] The Higgs-boson lineshape[428] The Higgs-boson lineshape[429] Heavy-quark mass effects in Higgs boson production at the LHC[429] Heavy-quark mass effects in Higgs boson production at the LHC[429] Heavy-quark mass effects in Higgs boson production at the LHC[429] Heavy-quark mass effects in Higgs boson production at the LHC[430] Evidence for the spin-0 nature of the Higgs boson using ATLAS data[430] Evidence for the spin-0 nature of the Higgs boson using ATLAS data[430] Evidence for the spin-0 nature of the Higgs boson using ATLAS data[430] Evidence for the spin-0 nature of the Higgs boson using ATLAS data[431] https://link.springer.com/content/pdf/10.1007/JHEP10(2013)222.pdf[431] https://link.springer.com/content/pdf/10.1007/JHEP10(2013)222.pdf[431] https://link.springer.com/content/pdf/10.1007/JHEP10(2013)222.pdf[431] https://link.springer.com/content/pdf/10.1007/JHEP10(2013)222.pdf[432] https://link.springer.com/content/pdf/10.1007/JHEP12(2013)089.pdf[432] https://link.springer.com/content/pdf/10.1007/JHEP12(2013)089.pdf[432] https://link.springer.com/content/pdf/10.1007/JHEP12(2013)089.pdf[432] https://link.springer.com/content/pdf/10.1007/JHEP12(2013)089.pdf[433] https://journals.aps.org/prd/pdf/10.1103/PhysRevD.89.092007[433] https://journals.aps.org/prd/pdf/10.1103/PhysRevD.89.092007[433] https://journals.aps.org/prd/pdf/10.1103/PhysRevD.89.092007[433] https://journals.aps.org/prd/pdf/10.1103/PhysRevD.89.092007[434] https://journals.aps.org/prl/pdf/10.1103/PhysRevLett.114.212001[434] https://journals.aps.org/prl/pdf/10.1103/PhysRevLett.114.212001[434] https://journals.aps.org/prl/pdf/10.1103/PhysRevLett.114.212001[434] https://journals.aps.org/prl/pdf/10.1103/PhysRevLett.114.212001[435] Lifetime and decay of "excited vacuum" states of a field theory associated with nonabsolute minima of its effective potential[435] Lifetime and decay of "excited vacuum" states of a field theory associated with nonabsolute minima of its effective potential[435] Lifetime and decay of "excited vacuum" states of a field theory associated with nonabsolute minima of its effective potential[435] Lifetime and decay of "excited vacuum" states of a field theory associated with nonabsolute minima of its effective potential[436] Semiclassical methods for unstable states[436] Semiclassical methods for unstable states[436] Semiclassical methods for unstable states[436] Semiclassical methods for unstable states[437] Consequences of vacuum instability in quantum field theory[437] Consequences of vacuum instability in quantum field theory[437] Consequences of vacuum instability in quantum field theory[437] Consequences of vacuum instability in quantum field theory[438] Fate of the false vacuum: Semiclassical theory[438] Fate of the false vacuum: Semiclassical theory[438] Fate of the false vacuum: Semiclassical theory[438] Fate of the false vacuum: Semiclassical theory[439] http://www.physics.princeton.edu/~steinh/ph564/Coleman.pdf[439] http://www.physics.princeton.edu/~steinh/ph564/Coleman.pdf[439] http://www.physics.princeton.edu/~steinh/ph564/Coleman.pdf[439] http://www.physics.princeton.edu/~steinh/ph564/Coleman.pdf[440] Fate of the false vacuum. II. First quantum corrections[440] Fate of the false vacuum. II. First quantum corrections[440] Fate of the false vacuum. II. First quantum corrections[440] Fate of the false vacuum. II. First quantum corrections[441] Vacuum Instability and Higgs Scalar Mass[441] Vacuum Instability and Higgs Scalar Mass[441] Vacuum Instability and Higgs Scalar Mass[441] Vacuum Instability and Higgs Scalar Mass[442] https://journals.aps.org/prd/pdf/10.1103/PhysRevD.23.347[442] https://journals.aps.org/prd/pdf/10.1103/PhysRevD.23.347[442] https://journals.aps.org/prd/pdf/10.1103/PhysRevD.23.347[442] https://journals.aps.org/prd/pdf/10.1103/PhysRevD.23.347[443] https://cds.cern.ch/record/133331/files/197907145.pdf[443] https://cds.cern.ch/record/133331/files/197907145.pdf[443] https://cds.cern.ch/record/133331/files/197907145.pdf[443] https://cds.cern.ch/record/133331/files/197907145.pdf[444] Is our vacuum metastable?[444] Is our vacuum metastable?[444] Is our vacuum metastable?[444] Is our vacuum metastable?[445] http://publish.uwo.ca/~csmeenk2/files/FalseVacuum.pdf[445] http://publish.uwo.ca/~csmeenk2/files/FalseVacuum.pdf[445] http://publish.uwo.ca/~csmeenk2/files/FalseVacuum.pdf[445] http://publish.uwo.ca/~csmeenk2/files/FalseVacuum.pdf[446] The top quark and Higgs boson masses and the stability of the electroweak vacuum[446] The top quark and Higgs boson masses and the stability of the electroweak vacuum[446] The top quark and Higgs boson masses and the stability of the electroweak vacuum[446] The top quark and Higgs boson masses and the stability of the electroweak vacuum[447] https://link.springer.com/content/pdf/10.1007/JHEP01(2015)061.pdf[447] https://link.springer.com/content/pdf/10.1007/JHEP01(2015)061.pdf[447] https://link.springer.com/content/pdf/10.1007/JHEP01(2015)061.pdf[447] https://link.springer.com/content/pdf/10.1007/JHEP01(2015)061.pdf[448] Electroweak vacuum instability and renormalized Higgs field vacuum fluctuations in the inflationary universe[448] Electroweak vacuum instability and renormalized Higgs field vacuum fluctuations in the inflationary universe[448] Electroweak vacuum instability and renormalized Higgs field vacuum fluctuations in the inflationary universe[448] Electroweak vacuum instability and renormalized Higgs field vacuum fluctuations in the inflationary universe[449] https://arxiv.org/pdf/1607.08133.pdf[449] https://arxiv.org/pdf/1607.08133.pdf[449] https://arxiv.org/pdf/1607.08133.pdf[449] https://arxiv.org/pdf/1607.08133.pdf[450] https://journals.aps.org/prd/pdf/10.1103/PhysRevD.98.103521[450] https://journals.aps.org/prd/pdf/10.1103/PhysRevD.98.103521[450] https://journals.aps.org/prd/pdf/10.1103/PhysRevD.98.103521[450] https://journals.aps.org/prd/pdf/10.1103/PhysRevD.98.103521[451] The probable fate of the Standard Model[451] The probable fate of the Standard Model[451] The probable fate of the Standard Model[451] The probable fate of the Standard Model[452] Higgs boson and top quark masses as tests of electroweak vacuum stability[452] Higgs boson and top quark masses as tests of electroweak vacuum stability[452] Higgs boson and top quark masses as tests of electroweak vacuum stability[452] Higgs boson and top quark masses as tests of electroweak vacuum stability[453] https://arxiv.org/pdf/1209.0393.pdf[453] https://arxiv.org/pdf/1209.0393.pdf[453] https://arxiv.org/pdf/1209.0393.pdf[453] https://arxiv.org/pdf/1209.0393.pdf[454] Cosmological Aspects of Higgs Vacuum Metastability[454] Cosmological Aspects of Higgs Vacuum Metastability[454] Cosmological Aspects of Higgs Vacuum Metastability[454] Cosmological Aspects of Higgs Vacuum Metastability[455] A simple motivated completion of the standard model below the Planck scale: Axions and right-handed neutrinos[455] A simple motivated completion of the standard model below the Planck scale: Axions and right-handed neutrinos[455] A simple motivated completion of the standard model below the Planck scale: Axions and right-handed neutrinos[455] A simple motivated completion of the standard model below the Planck scale: Axions and right-handed neutrinos[456] https://link.springer.com/content/pdf/10.1007/JHEP09(2014)182.pdf[456] https://link.springer.com/content/pdf/10.1007/JHEP09(2014)182.pdf[456] https://link.springer.com/content/pdf/10.1007/JHEP09(2014)182.pdf[456] https://link.springer.com/content/pdf/10.1007/JHEP09(2014)182.pdf[457] Vacuum metastability with black holes[457] Vacuum metastability with black holes[457] Vacuum metastability with black holes[457] Vacuum metastability with black holes[458] https://iopscience.iop.org/article/10.1088/1475-7516/2017/12/044[458] https://iopscience.iop.org/article/10.1088/1475-7516/2017/12/044[458] https://iopscience.iop.org/article/10.1088/1475-7516/2017/12/044[458] https://iopscience.iop.org/article/10.1088/1475-7516/2017/12/044[459] https://arxiv.org/pdf/1710.02865.pdf[459] https://arxiv.org/pdf/1710.02865.pdf[459] https://arxiv.org/pdf/1710.02865.pdf[459] https://arxiv.org/pdf/1710.02865.pdf[460] Vacuum decays around spinning black holes[460] Vacuum decays around spinning black holes[460] Vacuum decays around spinning black holes[460] Vacuum decays around spinning black holes[461] On the existence of $n$-geodesically complete or future complete solutions of Einstein's field equations with smooth asymptotic structure[461] On the existence of $n$-geodesically complete or future complete solutions of Einstein's field equations with smooth asymptotic structure[461] On the existence of $n$-geodesically complete or future complete solutions of Einstein's field equations with smooth asymptotic structure[461] On the existence of $n$-geodesically complete or future complete solutions of Einstein's field equations with smooth asymptotic structure[462] http://www.numdam.org/article/SEDP_1989-1990____A15_0.pdf[462] http://www.numdam.org/article/SEDP_1989-1990____A15_0.pdf[462] http://www.numdam.org/article/SEDP_1989-1990____A15_0.pdf[462] http://www.numdam.org/article/SEDP_1989-1990____A15_0.pdf[463] https://arxiv.org/pdf/1704.08681.pdf[463] https://arxiv.org/pdf/1704.08681.pdf[463] https://arxiv.org/pdf/1704.08681.pdf[463] https://arxiv.org/pdf/1704.08681.pdf[464] https://arxiv.org/pdf/1812.04268.pdf[464] https://arxiv.org/pdf/1812.04268.pdf[464] https://arxiv.org/pdf/1812.04268.pdf[464] https://arxiv.org/pdf/1812.04268.pdf[465] Black Holes Help Prove That a Special Kind of Space-Time Is Unstable[465] Black Holes Help Prove That a Special Kind of Space-Time Is Unstable[465] Black Holes Help Prove That a Special Kind of Space-Time Is Unstable[465] Black Holes Help Prove That a Special Kind of Space-Time Is Unstable[466] Gravitational effects on and of vacuum decay[466] Gravitational effects on and of vacuum decay[466] Gravitational effects on and of vacuum decay[466] Gravitational effects on and of vacuum decay[467] http://shortbus.org/x/p3305_1.pdf[467] http://shortbus.org/x/p3305_1.pdf[467] http://shortbus.org/x/p3305_1.pdf[467] http://shortbus.org/x/p3305_1.pdf[468] The End of Everything[468] The End of Everything[468] The End of Everything[468] The End of Everything[469] https://journals.aps.org/prl/pdf/10.1103/PhysRevLett.116.061102[469] https://journals.aps.org/prl/pdf/10.1103/PhysRevLett.116.061102[469] https://journals.aps.org/prl/pdf/10.1103/PhysRevLett.116.061102[469] https://journals.aps.org/prl/pdf/10.1103/PhysRevLett.116.061102[470] Gravitational Waves Discovered from Colliding Black Holes[470] Gravitational Waves Discovered from Colliding Black Holes[470] Gravitational Waves Discovered from Colliding Black Holes[470] Gravitational Waves Discovered from Colliding Black Holes[471] https://journals.aps.org/prl/pdf/10.1103/PhysRevLett.116.241103[471] https://journals.aps.org/prl/pdf/10.1103/PhysRevLett.116.241103[471] https://journals.aps.org/prl/pdf/10.1103/PhysRevLett.116.241103[471] https://journals.aps.org/prl/pdf/10.1103/PhysRevLett.116.241103[472] https://journals.aps.org/prl/pdf/10.1103/PhysRevLett.118.221101[472] https://journals.aps.org/prl/pdf/10.1103/PhysRevLett.118.221101[472] https://journals.aps.org/prl/pdf/10.1103/PhysRevLett.118.221101[472] https://journals.aps.org/prl/pdf/10.1103/PhysRevLett.118.221101[473] https://journals.aps.org/prl/pdf/10.1103/PhysRevLett.119.141101[473] https://journals.aps.org/prl/pdf/10.1103/PhysRevLett.119.141101[473] https://journals.aps.org/prl/pdf/10.1103/PhysRevLett.119.141101[473] https://journals.aps.org/prl/pdf/10.1103/PhysRevLett.119.141101[474] https://www.ligo.org/detections/GW170608/paper/GW170608_submitted.pdf[474] https://www.ligo.org/detections/GW170608/paper/GW170608_submitted.pdf[474] https://www.ligo.org/detections/GW170608/paper/GW170608_submitted.pdf[474] https://www.ligo.org/detections/GW170608/paper/GW170608_submitted.pdf[475] https://www.ligo.org/detections/O1O2catalog/o2catalog-submitted.pdf[475] https://www.ligo.org/detections/O1O2catalog/o2catalog-submitted.pdf[475] https://www.ligo.org/detections/O1O2catalog/o2catalog-submitted.pdf[475] https://www.ligo.org/detections/O1O2catalog/o2catalog-submitted.pdf[476] https://dcc.ligo.org/public/0163/P190412/009/gw190412-discovery.pdf[476] https://dcc.ligo.org/public/0163/P190412/009/gw190412-discovery.pdf[476] https://dcc.ligo.org/public/0163/P190412/009/gw190412-discovery.pdf[476] https://dcc.ligo.org/public/0163/P190412/009/gw190412-discovery.pdf[477] https://journals.aps.org/prl/pdf/10.1103/PhysRevLett.119.161101[477] https://journals.aps.org/prl/pdf/10.1103/PhysRevLett.119.161101[477] https://journals.aps.org/prl/pdf/10.1103/PhysRevLett.119.161101[477] https://journals.aps.org/prl/pdf/10.1103/PhysRevLett.119.161101[478] https://dcc.ligo.org/public/0161/P190425/009/Abbott_2020_ApJL_892_L3.pdf[478] https://dcc.ligo.org/public/0161/P190425/009/Abbott_2020_ApJL_892_L3.pdf[478] https://dcc.ligo.org/public/0161/P190425/009/Abbott_2020_ApJL_892_L3.pdf[478] https://dcc.ligo.org/public/0161/P190425/009/Abbott_2020_ApJL_892_L3.pdf[479] ShieldSquare Captcha[479] ShieldSquare Captcha[479] ShieldSquare Captcha[479] ShieldSquare Captcha[480] Mystery Object Blurs Line between Neutron Stars and Black Holes[480] Mystery Object Blurs Line between Neutron Stars and Black Holes[480] Mystery Object Blurs Line between Neutron Stars and Black Holes[480] Mystery Object Blurs Line between Neutron Stars and Black Holes[481] Relativistic Shapiro delay measurements of an extremely massive millisecond pulsar[481] Relativistic Shapiro delay measurements of an extremely massive millisecond pulsar[481] Relativistic Shapiro delay measurements of an extremely massive millisecond pulsar[481] Relativistic Shapiro delay measurements of an extremely massive millisecond pulsar[482] Relativistic Shapiro delay measurements of an extremely massive millisecond pulsar[482] Relativistic Shapiro delay measurements of an extremely massive millisecond pulsar[482] Relativistic Shapiro delay measurements of an extremely massive millisecond pulsar[482] Relativistic Shapiro delay measurements of an extremely massive millisecond pulsar[483] A noninteracting low-mass black hole–giant star binary system[483] A noninteracting low-mass black hole–giant star binary system[483] A noninteracting low-mass black hole–giant star binary system[483] A noninteracting low-mass black hole–giant star binary system[484] https://arxiv.org/pdf/1806.02751.pdf[484] https://arxiv.org/pdf/1806.02751.pdf[484] https://arxiv.org/pdf/1806.02751.pdf[484] https://arxiv.org/pdf/1806.02751.pdf[485] Hypothesis concerning quark stars[485] Hypothesis concerning quark stars[485] Hypothesis concerning quark stars[485] Hypothesis concerning quark stars[486] Remarks on quark stars[486] Remarks on quark stars[486] Remarks on quark stars[486] Remarks on quark stars[487] https://cds.cern.ch/record/278675/files/SCAN-9503155.pdf[487] https://cds.cern.ch/record/278675/files/SCAN-9503155.pdf[487] https://cds.cern.ch/record/278675/files/SCAN-9503155.pdf[487] https://cds.cern.ch/record/278675/files/SCAN-9503155.pdf[488] https://arxiv.org/pdf/2007.03799.pdf[488] https://arxiv.org/pdf/2007.03799.pdf[488] https://arxiv.org/pdf/2007.03799.pdf[488] https://arxiv.org/pdf/2007.03799.pdf[489] LHCb Experiment Discovers CP Violation in the Charm Quark System[489] LHCb Experiment Discovers CP Violation in the Charm Quark System[489] LHCb Experiment Discovers CP Violation in the Charm Quark System[489] LHCb Experiment Discovers CP Violation in the Charm Quark System[490] LHCb discovers matter-antimatter asymmetry in charm quarks[490] LHCb discovers matter-antimatter asymmetry in charm quarks[490] LHCb discovers matter-antimatter asymmetry in charm quarks[490] LHCb discovers matter-antimatter asymmetry in charm quarks[491] Searching for matter–antimatter asymmetry in the Higgs boson–top quark interaction[491] Searching for matter–antimatter asymmetry in the Higgs boson–top quark interaction[491] Searching for matter–antimatter asymmetry in the Higgs boson–top quark interaction[491] Searching for matter–antimatter asymmetry in the Higgs boson–top quark interaction[492] Neutrino Asymmetry Passes Critical Threshold[492] Neutrino Asymmetry Passes Critical Threshold[492] Neutrino Asymmetry Passes Critical Threshold[492] Neutrino Asymmetry Passes Critical Threshold[493] Closing in on matter-antimatter asymmetry: T2K results restrict possible values of neutrino CP phase[493] Closing in on matter-antimatter asymmetry: T2K results restrict possible values of neutrino CP phase[493] Closing in on matter-antimatter asymmetry: T2K results restrict possible values of neutrino CP phase[493] Closing in on matter-antimatter asymmetry: T2K results restrict possible values of neutrino CP phase[494] https://research.ipmu.jp/ipmu/sysimg/ipmu/1632.pdf[494] https://research.ipmu.jp/ipmu/sysimg/ipmu/1632.pdf[494] https://research.ipmu.jp/ipmu/sysimg/ipmu/1632.pdf[494] https://research.ipmu.jp/ipmu/sysimg/ipmu/1632.pdf[495] A Strange New Higgs Particle May Have Stolen the Antimatter from Our Universe[495] A Strange New Higgs Particle May Have Stolen the Antimatter from Our Universe[495] A Strange New Higgs Particle May Have Stolen the Antimatter from Our Universe[495] A Strange New Higgs Particle May Have Stolen the Antimatter from Our Universe[496] https://arxiv.org/pdf/1909.02044.pdf[496] https://arxiv.org/pdf/1909.02044.pdf[496] https://arxiv.org/pdf/1909.02044.pdf[496] https://arxiv.org/pdf/1909.02044.pdf[497] https://arxiv.org/pdf/1408.1811.pdf[497] https://arxiv.org/pdf/1408.1811.pdf[497] https://arxiv.org/pdf/1408.1811.pdf[497] https://arxiv.org/pdf/1408.1811.pdf[498] https://arxiv.org/pdf/1106.5408.pdf[498] https://arxiv.org/pdf/1106.5408.pdf[498] https://arxiv.org/pdf/1106.5408.pdf[498] https://arxiv.org/pdf/1106.5408.pdf[499] Primordial black holes and the origin of the matter-antimatter asymmetry[499] Primordial black holes and the origin of the matter-antimatter asymmetry[499] Primordial black holes and the origin of the matter-antimatter asymmetry[499] Primordial black holes and the origin of the matter-antimatter asymmetry[500] http://physics.princeton.edu/~steinh/BlackHoleBaryo.pdf[500] http://physics.princeton.edu/~steinh/BlackHoleBaryo.pdf[500] http://physics.princeton.edu/~steinh/BlackHoleBaryo.pdf[500] http://physics.princeton.edu/~steinh/BlackHoleBaryo.pdf[501] Physicists propose mechanism that explains the origins of both dark matter and 'normal' matter[501] Physicists propose mechanism that explains the origins of both dark matter and 'normal' matter[501] Physicists propose mechanism that explains the origins of both dark matter and 'normal' matter[501] Physicists propose mechanism that explains the origins of both dark matter and 'normal' matter[502] https://arxiv.org/pdf/1101.4012.pdf[502] https://arxiv.org/pdf/1101.4012.pdf[502] https://arxiv.org/pdf/1101.4012.pdf[502] https://arxiv.org/pdf/1101.4012.pdf[503] Observation of excess events in the XENON1T dark matter experiment[503] Observation of excess events in the XENON1T dark matter experiment[503] Observation of excess events in the XENON1T dark matter experiment[503] Observation of excess events in the XENON1T dark matter experiment[504] Dark Matter Experiment Finds Unexplained Signal[504] Dark Matter Experiment Finds Unexplained Signal[504] Dark Matter Experiment Finds Unexplained Signal[504] Dark Matter Experiment Finds Unexplained Signal[505] Seeking Dark Matter, They Detected Another Mystery[505] Seeking Dark Matter, They Detected Another Mystery[505] Seeking Dark Matter, They Detected Another Mystery[505] Seeking Dark Matter, They Detected Another Mystery[506] Dark matter hunt yields unexplained signal[506] Dark matter hunt yields unexplained signal[506] Dark matter hunt yields unexplained signal[506] Dark matter hunt yields unexplained signal[507] Physicists Announce Potential Dark Matter Breakthrough[507] Physicists Announce Potential Dark Matter Breakthrough[507] Physicists Announce Potential Dark Matter Breakthrough[507] Physicists Announce Potential Dark Matter Breakthrough[508] https://arxiv.org/pdf/2006.09721.pdf[508] https://arxiv.org/pdf/2006.09721.pdf[508] https://arxiv.org/pdf/2006.09721.pdf[508] https://arxiv.org/pdf/2006.09721.pdf[509] Is Dark Matter Made of Axions?[509] Is Dark Matter Made of Axions?[509] Is Dark Matter Made of Axions?[509] Is Dark Matter Made of Axions?[510] Case for axion origin of dark matter gains traction[510] Case for axion origin of dark matter gains traction[510] Case for axion origin of dark matter gains traction[510] Case for axion origin of dark matter gains traction[511] https://journals.aps.org/prl/pdf/10.1103/PhysRevLett.124.251802[511] https://journals.aps.org/prl/pdf/10.1103/PhysRevLett.124.251802[511] https://journals.aps.org/prl/pdf/10.1103/PhysRevLett.124.251802[511] https://journals.aps.org/prl/pdf/10.1103/PhysRevLett.124.251802[512] https://indico.cern.ch/event/427023/contributions/1050624/attachments/912026/1288208/Lancester-Mannel-Proc.pdf[512] https://indico.cern.ch/event/427023/contributions/1050624/attachments/912026/1288208/Lancester-Mannel-Proc.pdf[512] https://indico.cern.ch/event/427023/contributions/1050624/attachments/912026/1288208/Lancester-Mannel-Proc.pdf[512] https://indico.cern.ch/event/427023/contributions/1050624/attachments/912026/1288208/Lancester-Mannel-Proc.pdf[513] $\mathrm{CP}$[513] $\mathrm{CP}$[513] $\mathrm{CP}$[513] $\mathrm{CP}$[514] Axions and Family Symmetry Breaking[514] Axions and Family Symmetry Breaking[514] Axions and Family Symmetry Breaking[514] Axions and Family Symmetry Breaking[515] Weak-Interaction Singlet and Strong $\mathrm{CP}$ Invariance[515] Weak-Interaction Singlet and Strong $\mathrm{CP}$ Invariance[515] Weak-Interaction Singlet and Strong $\mathrm{CP}$ Invariance[515] Weak-Interaction Singlet and Strong $\mathrm{CP}$ Invariance[516] Can confinement ensure natural CP invariance of strong interactions?[516] Can confinement ensure natural CP invariance of strong interactions?[516] Can confinement ensure natural CP invariance of strong interactions?[516] Can confinement ensure natural CP invariance of strong interactions?[517] A simple solution to the strong CP problem with a harmless axion[517] A simple solution to the strong CP problem with a harmless axion[517] A simple solution to the strong CP problem with a harmless axion[517] A simple solution to the strong CP problem with a harmless axion[518] http://www.theory.caltech.edu/~preskill/pubs/preskill-1983-axion.pdf[518] http://www.theory.caltech.edu/~preskill/pubs/preskill-1983-axion.pdf[518] http://www.theory.caltech.edu/~preskill/pubs/preskill-1983-axion.pdf[518] http://www.theory.caltech.edu/~preskill/pubs/preskill-1983-axion.pdf[519] A cosmological bound on the invisible axion[519] A cosmological bound on the invisible axion[519] A cosmological bound on the invisible axion[519] A cosmological bound on the invisible axion[520] The not-so-harmless axion[520] The not-so-harmless axion[520] The not-so-harmless axion[520] The not-so-harmless axion[521] Calculation of the axion mass based on high-temperature lattice quantum chromodynamics[521] Calculation of the axion mass based on high-temperature lattice quantum chromodynamics[521] Calculation of the axion mass based on high-temperature lattice quantum chromodynamics[521] Calculation of the axion mass based on high-temperature lattice quantum chromodynamics[522] Search for axion-like dark matter with ferromagnets[522] Search for axion-like dark matter with ferromagnets[522] Search for axion-like dark matter with ferromagnets[522] Search for axion-like dark matter with ferromagnets[523] https://arxiv.org/pdf/2003.03348.pdf[523] https://arxiv.org/pdf/2003.03348.pdf[523] https://arxiv.org/pdf/2003.03348.pdf[523] https://arxiv.org/pdf/2003.03348.pdf[524] Evidence Builds for a New Kind of Neutrino[524] Evidence Builds for a New Kind of Neutrino[524] Evidence Builds for a New Kind of Neutrino[524] Evidence Builds for a New Kind of Neutrino[525] An Alternative Analysis of the LSND Neutrino Oscillation Search Data on ${\overline{\ensuremath{\nu}}}_{\ensuremath{\mu}}\ensuremath{\rightarrow}{\overline{\ensuremath{\nu}}}_{\mathit{e}}$[525] An Alternative Analysis of the LSND Neutrino Oscillation Search Data on ${\overline{\ensuremath{\nu}}}_{\ensuremath{\mu}}\ensuremath{\rightarrow}{\overline{\ensuremath{\nu}}}_{\mathit{e}}$[525] An Alternative Analysis of the LSND Neutrino Oscillation Search Data on ${\overline{\ensuremath{\nu}}}_{\ensuremath{\mu}}\ensuremath{\rightarrow}{\overline{\ensuremath{\nu}}}_{\mathit{e}}$[525] An Alternative Analysis of the LSND Neutrino Oscillation Search Data on ${\overline{\ensuremath{\nu}}}_{\ensuremath{\mu}}\ensuremath{\rightarrow}{\overline{\ensuremath{\nu}}}_{\mathit{e}}$[526] https://arxiv.org/pdf/hep-ex/9504009.pdf[526] https://arxiv.org/pdf/hep-ex/9504009.pdf[526] https://arxiv.org/pdf/hep-ex/9504009.pdf[526] https://arxiv.org/pdf/hep-ex/9504009.pdf[527] The liquid scintillator neutrino detector and LAMPF neutrino source[527] The liquid scintillator neutrino detector and LAMPF neutrino source[527] The liquid scintillator neutrino detector and LAMPF neutrino source[527] The liquid scintillator neutrino detector and LAMPF neutrino source[528] https://arxiv.org/pdf/nucl-ex/9605002.pdf[528] https://arxiv.org/pdf/nucl-ex/9605002.pdf[528] https://arxiv.org/pdf/nucl-ex/9605002.pdf[528] https://arxiv.org/pdf/nucl-ex/9605002.pdf[529] https://journals.aps.org/prl/pdf/10.1103/PhysRevLett.121.221801[529] https://journals.aps.org/prl/pdf/10.1103/PhysRevLett.121.221801[529] https://journals.aps.org/prl/pdf/10.1103/PhysRevLett.121.221801[529] https://journals.aps.org/prl/pdf/10.1103/PhysRevLett.121.221801[530] Hidden Neutrino Particles May Be a Link to the Dark Sector[530] Hidden Neutrino Particles May Be a Link to the Dark Sector[530] Hidden Neutrino Particles May Be a Link to the Dark Sector[530] Hidden Neutrino Particles May Be a Link to the Dark Sector[531] http://downloads.hindawi.com/journals/ahep/2012/718259.pdf[531] http://downloads.hindawi.com/journals/ahep/2012/718259.pdf[531] http://downloads.hindawi.com/journals/ahep/2012/718259.pdf[531] http://downloads.hindawi.com/journals/ahep/2012/718259.pdf[532] https://journals.aps.org/prl/pdf/10.1103/PhysRevLett.125.071801[532] https://journals.aps.org/prl/pdf/10.1103/PhysRevLett.125.071801[532] https://journals.aps.org/prl/pdf/10.1103/PhysRevLett.125.071801[532] https://journals.aps.org/prl/pdf/10.1103/PhysRevLett.125.071801[533] https://www.quantamagazine.org/the-black-hole-information-paradox-comes-to-an-end-20201029/[533] https://www.quantamagazine.org/the-black-hole-information-paradox-comes-to-an-end-20201029/[533] https://www.quantamagazine.org/the-black-hole-information-paradox-comes-to-an-end-20201029/[533] https://www.quantamagazine.org/the-black-hole-information-paradox-comes-to-an-end-20201029/[534] Information in black hole radiation[534] Information in black hole radiation[534] Information in black hole radiation[534] Information in black hole radiation[535] https://arxiv.org/pdf/hep-th/9305040.pdf[535] https://arxiv.org/pdf/hep-th/9305040.pdf[535] https://arxiv.org/pdf/hep-th/9305040.pdf[535] https://arxiv.org/pdf/hep-th/9305040.pdf[536] Is Black-Hole Evaporation Predictable?[536] Is Black-Hole Evaporation Predictable?[536] Is Black-Hole Evaporation Predictable?[536] Is Black-Hole Evaporation Predictable?[537] https://arxiv.org/pdf/1810.02055.pdf[537] https://arxiv.org/pdf/1810.02055.pdf[537] https://arxiv.org/pdf/1810.02055.pdf[537] https://arxiv.org/pdf/1810.02055.pdf[538] https://arxiv.org/pdf/1905.08762.pdf[538] https://arxiv.org/pdf/1905.08762.pdf[538] https://arxiv.org/pdf/1905.08762.pdf[538] https://arxiv.org/pdf/1905.08762.pdf[539] https://arxiv.org/pdf/1905.08255.pdf[539] https://arxiv.org/pdf/1905.08255.pdf[539] https://arxiv.org/pdf/1905.08255.pdf[539] https://arxiv.org/pdf/1905.08255.pdf[540] https://arxiv.org/pdf/0804.0055.pdf[540] https://arxiv.org/pdf/0804.0055.pdf[540] https://arxiv.org/pdf/0804.0055.pdf[540] https://arxiv.org/pdf/0804.0055.pdf[541] https://arxiv.org/pdf/1408.3203.pdf[541] https://arxiv.org/pdf/1408.3203.pdf[541] https://arxiv.org/pdf/1408.3203.pdf[541] https://arxiv.org/pdf/1408.3203.pdf[542] https://arxiv.org/pdf/1905.08762.pdf[542] https://arxiv.org/pdf/1905.08762.pdf[542] https://arxiv.org/pdf/1905.08762.pdf[542] https://arxiv.org/pdf/1905.08762.pdf[543] https://arxiv.org/pdf/2004.05863.pdf[543] https://arxiv.org/pdf/2004.05863.pdf[543] https://arxiv.org/pdf/2004.05863.pdf[543] https://arxiv.org/pdf/2004.05863.pdf[544] https://arxiv.org/pdf/1908.10996.pdf[544] https://arxiv.org/pdf/1908.10996.pdf[544] https://arxiv.org/pdf/1908.10996.pdf[544] https://arxiv.org/pdf/1908.10996.pdf[545] Hologram Within a Hologram Hints at Fate of Black Holes[545] Hologram Within a Hologram Hints at Fate of Black Holes[545] Hologram Within a Hologram Hints at Fate of Black Holes[545] Hologram Within a Hologram Hints at Fate of Black Holes[546] https://arxiv.org/pdf/1911.11977.pdf[546] https://arxiv.org/pdf/1911.11977.pdf[546] https://arxiv.org/pdf/1911.11977.pdf[546] https://arxiv.org/pdf/1911.11977.pdf[547] https://arxiv.org/pdf/1911.12333.pdf[547] https://arxiv.org/pdf/1911.12333.pdf[547] https://arxiv.org/pdf/1911.12333.pdf[547] https://arxiv.org/pdf/1911.12333.pdf[548] https://arxiv.org/pdf/1306.0533.pdf[548] https://arxiv.org/pdf/1306.0533.pdf[548] https://arxiv.org/pdf/1306.0533.pdf[548] https://arxiv.org/pdf/1306.0533.pdf[549] https://arxiv.org/pdf/hep-th/0509010.pdf[549] https://arxiv.org/pdf/hep-th/0509010.pdf[549] https://arxiv.org/pdf/hep-th/0509010.pdf[549] https://arxiv.org/pdf/hep-th/0509010.pdf[550] In Violation of Einstein, Black Holes Might Have ‘Hair’[550] In Violation of Einstein, Black Holes Might Have ‘Hair’[550] In Violation of Einstein, Black Holes Might Have ‘Hair’[550] In Violation of Einstein, Black Holes Might Have ‘Hair’[551] https://arxiv.org/pdf/1206.6598.pdf[551] https://arxiv.org/pdf/1206.6598.pdf[551] https://arxiv.org/pdf/1206.6598.pdf[551] https://arxiv.org/pdf/1206.6598.pdf[552] Horizon Hair of Extremal Black Holes and Measurements at Null Infinity[552] Horizon Hair of Extremal Black Holes and Measurements at Null Infinity[552] Horizon Hair of Extremal Black Holes and Measurements at Null Infinity[552] Horizon Hair of Extremal Black Holes and Measurements at Null Infinity[553] Scalar and gravitational hair for extreme Kerr black holes[553] Scalar and gravitational hair for extreme Kerr black holes[553] Scalar and gravitational hair for extreme Kerr black holes[553] Scalar and gravitational hair for extreme Kerr black holes[554] https://arxiv.org/pdf/0704.2276.pdf[554] https://arxiv.org/pdf/0704.2276.pdf[554] https://arxiv.org/pdf/0704.2276.pdf[554] https://arxiv.org/pdf/0704.2276.pdf[555] https://arxiv.org/pdf/1005.1676.pdf[555] https://arxiv.org/pdf/1005.1676.pdf[555] https://arxiv.org/pdf/1005.1676.pdf[555] https://arxiv.org/pdf/1005.1676.pdf[556] Quantum gravity: general introduction and recent developments[556] Quantum gravity: general introduction and recent developments[556] Quantum gravity: general introduction and recent developments[556] Quantum gravity: general introduction and recent developments[557] https://arxiv.org/pdf/gr-qc/0508120.pdf[557] https://arxiv.org/pdf/gr-qc/0508120.pdf[557] https://arxiv.org/pdf/gr-qc/0508120.pdf[557] https://arxiv.org/pdf/gr-qc/0508120.pdf[558] Black Hole Singularities Are as Inescapable as Expected | Quanta Magazine[558] Black Hole Singularities Are as Inescapable as Expected | Quanta Magazine[558] Black Hole Singularities Are as Inescapable as Expected | Quanta Magazine[558] Black Hole Singularities Are as Inescapable as Expected | Quanta Magazine[559] AXION-INDUCED TOPOLOGY CHANGE IN QUANTUM GRAVITY AND STRING THEORY[559] AXION-INDUCED TOPOLOGY CHANGE IN QUANTUM GRAVITY AND STRING THEORY[559] AXION-INDUCED TOPOLOGY CHANGE IN QUANTUM GRAVITY AND STRING THEORY[559] AXION-INDUCED TOPOLOGY CHANGE IN QUANTUM GRAVITY AND STRING THEORY[560] http://citeseerx.ist.psu.edu/viewdoc/download?doi=10.1.1.216.7417&rep=rep1&type=pdf[560] http://citeseerx.ist.psu.edu/viewdoc/download?doi=10.1.1.216.7417&rep=rep1&type=pdf[560] http://citeseerx.ist.psu.edu/viewdoc/download?doi=10.1.1.216.7417&rep=rep1&type=pdf[560] http://citeseerx.ist.psu.edu/viewdoc/download?doi=10.1.1.216.7417&rep=rep1&type=pdf[561] Fermionic string field theory of c = 1 two-dimensional quantum gravity[561] Fermionic string field theory of c = 1 two-dimensional quantum gravity[561] Fermionic string field theory of c = 1 two-dimensional quantum gravity[561] Fermionic string field theory of c = 1 two-dimensional quantum gravity[562] A generalized uncertainty principle in quantum gravity[562] A generalized uncertainty principle in quantum gravity[562] A generalized uncertainty principle in quantum gravity[562] A generalized uncertainty principle in quantum gravity[563] https://arxiv.org/pdf/hep-th/9301067.pdf[563] https://arxiv.org/pdf/hep-th/9301067.pdf[563] https://arxiv.org/pdf/hep-th/9301067.pdf[563] https://arxiv.org/pdf/hep-th/9301067.pdf[564] https://iopscience.iop.org/article/10.1088/1126-6708/1999/01/007/pdf[564] https://iopscience.iop.org/article/10.1088/1126-6708/1999/01/007/pdf[564] https://iopscience.iop.org/article/10.1088/1126-6708/1999/01/007/pdf[564] https://iopscience.iop.org/article/10.1088/1126-6708/1999/01/007/pdf[565] Locality in quantum gravity and string theory[565] Locality in quantum gravity and string theory[565] Locality in quantum gravity and string theory[565] Locality in quantum gravity and string theory[566] https://arxiv.org/pdf/hep-th/0604072.pdf[566] https://arxiv.org/pdf/hep-th/0604072.pdf[566] https://arxiv.org/pdf/hep-th/0604072.pdf[566] https://arxiv.org/pdf/hep-th/0604072.pdf[567] https://www.worldscientific.com/doi/pdf/10.1142/S0218271814420061[567] https://www.worldscientific.com/doi/pdf/10.1142/S0218271814420061[567] https://www.worldscientific.com/doi/pdf/10.1142/S0218271814420061[567] https://www.worldscientific.com/doi/pdf/10.1142/S0218271814420061[568] Two-loop quantum gravity[568] Two-loop quantum gravity[568] Two-loop quantum gravity[568] Two-loop quantum gravity[569] Black Hole Entropy from Loop Quantum Gravity[569] Black Hole Entropy from Loop Quantum Gravity[569] Black Hole Entropy from Loop Quantum Gravity[569] Black Hole Entropy from Loop Quantum Gravity[570] https://arxiv.org/pdf/gr-qc/9603063.pdf[570] https://arxiv.org/pdf/gr-qc/9603063.pdf[570] https://arxiv.org/pdf/gr-qc/9603063.pdf[570] https://arxiv.org/pdf/gr-qc/9603063.pdf[571] ``Sum over surfaces'' form of loop quantum gravity[571] ``Sum over surfaces'' form of loop quantum gravity[571] ``Sum over surfaces'' form of loop quantum gravity[571] ``Sum over surfaces'' form of loop quantum gravity[572] https://arxiv.org/pdf/gr-qc/9612035.pdf[572] https://arxiv.org/pdf/gr-qc/9612035.pdf[572] https://arxiv.org/pdf/gr-qc/9612035.pdf[572] https://arxiv.org/pdf/gr-qc/9612035.pdf[573] Loop quantum gravity and light propagation[573] Loop quantum gravity and light propagation[573] Loop quantum gravity and light propagation[573] Loop quantum gravity and light propagation[574] https://arxiv.org/pdf/hep-th/0108061.pdf[574] https://arxiv.org/pdf/hep-th/0108061.pdf[574] https://arxiv.org/pdf/hep-th/0108061.pdf[574] https://arxiv.org/pdf/hep-th/0108061.pdf[575] Lectures on Loop Quantum Gravity[575] Lectures on Loop Quantum Gravity[575] Lectures on Loop Quantum Gravity[575] Lectures on Loop Quantum Gravity[576] https://arxiv.org/pdf/gr-qc/0210094.pdf[576] https://arxiv.org/pdf/gr-qc/0210094.pdf[576] https://arxiv.org/pdf/gr-qc/0210094.pdf[576] https://arxiv.org/pdf/gr-qc/0210094.pdf[577] https://iopscience.iop.org/article/10.1088/0264-9381/21/22/015/meta[577] https://iopscience.iop.org/article/10.1088/0264-9381/21/22/015/meta[577] https://iopscience.iop.org/article/10.1088/0264-9381/21/22/015/meta[577] https://iopscience.iop.org/article/10.1088/0264-9381/21/22/015/meta[578] https://arxiv.org/pdf/gr-qc/0407052.pdf[578] https://arxiv.org/pdf/gr-qc/0407052.pdf[578] https://arxiv.org/pdf/gr-qc/0407052.pdf[578] https://arxiv.org/pdf/gr-qc/0407052.pdf[579] Loop-Quantum-Gravity Vertex Amplitude[579] Loop-Quantum-Gravity Vertex Amplitude[579] Loop-Quantum-Gravity Vertex Amplitude[579] Loop-Quantum-Gravity Vertex Amplitude[580] https://arxiv.org/pdf/0705.2388.pdf[580] https://arxiv.org/pdf/0705.2388.pdf[580] https://arxiv.org/pdf/0705.2388.pdf[580] https://arxiv.org/pdf/0705.2388.pdf[581] https://link.springer.com/content/pdf/10.12942/lrr-2008-5.pdf[581] https://link.springer.com/content/pdf/10.12942/lrr-2008-5.pdf[581] https://link.springer.com/content/pdf/10.12942/lrr-2008-5.pdf[581] https://link.springer.com/content/pdf/10.12942/lrr-2008-5.pdf[582] Polyhedra in loop quantum gravity[582] Polyhedra in loop quantum gravity[582] Polyhedra in loop quantum gravity[582] Polyhedra in loop quantum gravity[583] https://arxiv.org/pdf/1009.3402.pdf[583] https://arxiv.org/pdf/1009.3402.pdf[583] https://arxiv.org/pdf/1009.3402.pdf[583] https://arxiv.org/pdf/1009.3402.pdf[584] Unity of All Elementary-Particle Forces[584] Unity of All Elementary-Particle Forces[584] Unity of All Elementary-Particle Forces[584] Unity of All Elementary-Particle Forces[585] Aspects of the grand unification of strong, weak and electromagnetic interactions[585] Aspects of the grand unification of strong, weak and electromagnetic interactions[585] Aspects of the grand unification of strong, weak and electromagnetic interactions[585] Aspects of the grand unification of strong, weak and electromagnetic interactions[586] http://cdsweb.cern.ch/record/132734/files/197712054.pdf[586] http://cdsweb.cern.ch/record/132734/files/197712054.pdf[586] http://cdsweb.cern.ch/record/132734/files/197712054.pdf[586] http://cdsweb.cern.ch/record/132734/files/197712054.pdf[587] https://arxiv.org/ftp/gr-qc/papers/9910/9910036.pdf[587] https://arxiv.org/ftp/gr-qc/papers/9910/9910036.pdf[587] https://arxiv.org/ftp/gr-qc/papers/9910/9910036.pdf[587] https://arxiv.org/ftp/gr-qc/papers/9910/9910036.pdf[588] WMAP- Fate of the Universe[588] WMAP- Fate of the Universe[588] WMAP- Fate of the Universe[588] WMAP- Fate of the Universe[589] Space and time in the quantum universe.[589] Space and time in the quantum universe.[589] Space and time in the quantum universe.[589] Space and time in the quantum universe.[590] Thermodynamics in the viscous early universe[590] Thermodynamics in the viscous early universe[590] Thermodynamics in the viscous early universe[590] Thermodynamics in the viscous early universe[591] https://arxiv.org/pdf/1002.0296.pdf[591] https://arxiv.org/pdf/1002.0296.pdf[591] https://arxiv.org/pdf/1002.0296.pdf[591] https://arxiv.org/pdf/1002.0296.pdf[592] Thermodynamics of viscous matter and radiation in the early universe[592] Thermodynamics of viscous matter and radiation in the early universe[592] Thermodynamics of viscous matter and radiation in the early universe[592] Thermodynamics of viscous matter and radiation in the early universe[593] https://arxiv.org/pdf/1109.6469.pdf[593] https://arxiv.org/pdf/1109.6469.pdf[593] https://arxiv.org/pdf/1109.6469.pdf[593] https://arxiv.org/pdf/1109.6469.pdf[594] Is emergent universe a consequence of particle creation process?[594] Is emergent universe a consequence of particle creation process?[594] Is emergent universe a consequence of particle creation process?[594] Is emergent universe a consequence of particle creation process?[595] Structure of Relatively Accelerating Universe[595] Structure of Relatively Accelerating Universe[595] Structure of Relatively Accelerating Universe[595] Structure of Relatively Accelerating Universe
- Home >
- Catalog >
- Business >
- Ticket Template >
- Exit Ticket Template >
- Determine Whether The Ratios Are Equivalent 3 10 And 6 20